Abstract
Human rehabilitation improved significantly after traumas, surgery, or accidental cross-link events with human health. During the last six decades, exoskeletons have played a significant role in human activities related to body training and post-trauma or surgery treatment, especially in gait rehabilitation. The main goal of rehabilitation training is to restore patients’ physical abilities to average by improving and monitoring their posture and gaining weight. In this paper, a classification of various types of exoskeletons is provided, a comparison between the different lower limb exoskeletons for gait rehabilitation presents, the gait anatomy, mechanical design, and control strategy for the prototype of lower limb exoskeleton studies, and the end, some concluding remarks are stated that may be useful for future work. The paper concludes with conclusions and a significant reference list.
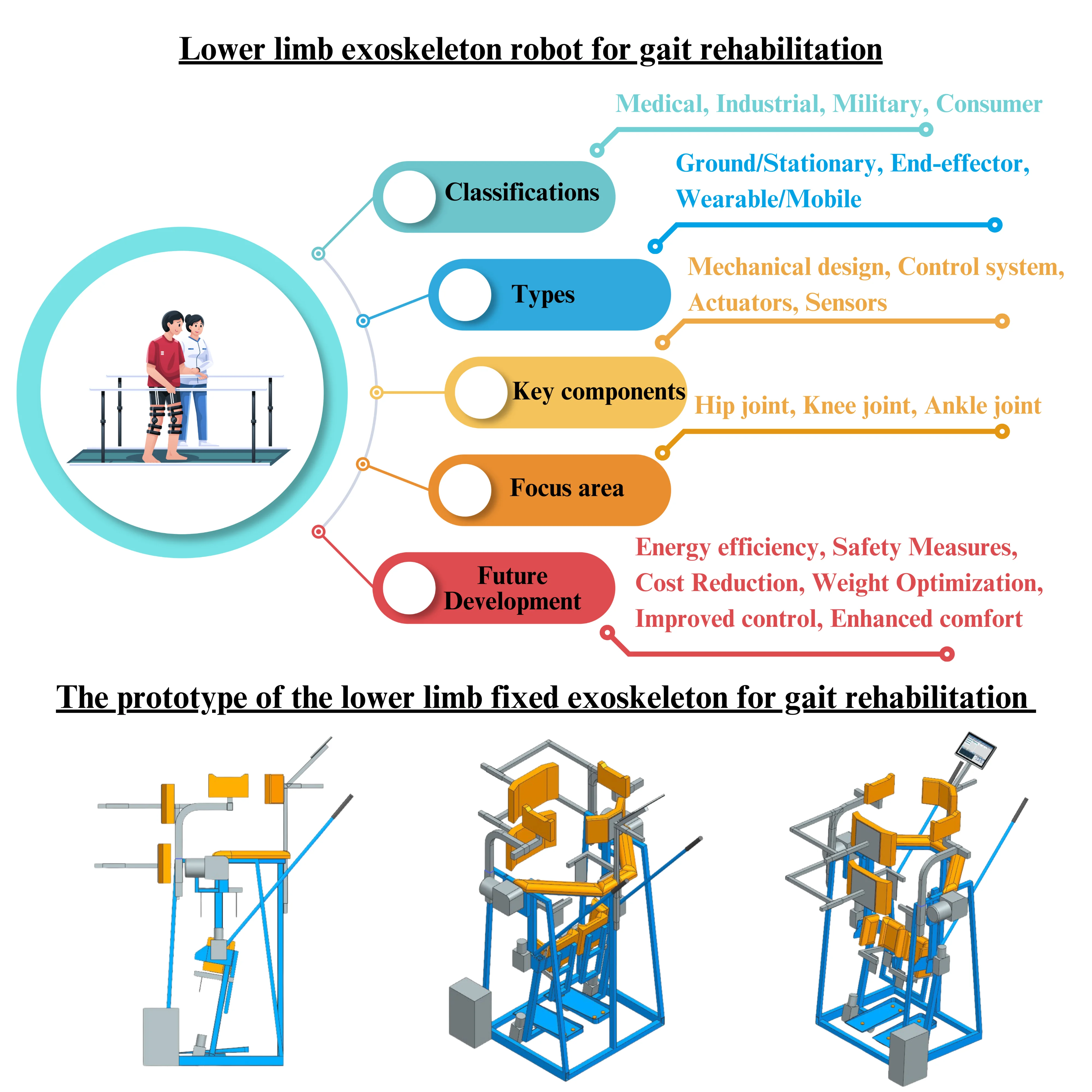
Highlights
- Identify the Current State of Medical Robotics and the tasks they can perform.
- Identify the Classification of Medical Exoskeletons according to the purpose of use, the principle of operation, power source, mechanical design, and other factors.
- Analysis of Commercial Fixed-Frame Systems based on body area, DOF, Control system, power type, actuators, and sensors. Detailed comparison of five major systems: Lokomat, ReoAmbulator, Walkbot, MRG-P110, and Gait Simulator.
- Technical Design Considerations: Gait anatomy, mechanical design, control structure, actuators, and sensors.
- Identify the Future Research Directions: Energy efficiency, Lower costs, Lower weight, and Human-Robot Collaboration.
1. Introduction
Robots are ubiquitous devices widely used in industries ranging from manufacturing to construction to medical facilities. Extensive research on robots is being conducted in higher education and scientific research institutes. They are exploring new applications for robots and robotic systems and how they can improve society and help people. Robots in medicine are leading to significant advances, and robots help patients with load augmentation, trauma patients, paraplegics, and spinal cord-injured people for rehabilitation purposes [1]. The global market for medical robotics is anticipated to grow significantly, with projections estimating its value to reach around $12.7 billion by 2025. This growth represents a significant advancement in integrating robotics within the healthcare sector, enhancing surgical precision, streamlining hospital workflows, and improving patient outcomes through innovative technological solutions. In 2020, hospital robot adoption accounted for the largest market share, and the integration of robotics within the healthcare sector continues to grow at an impressive rate [2].
Various medical robots are thoughtfully developed to carry out specific functions [3]. Modular robots, also known as exoskeleton robots, are essential in the rehabilitation of individuals recovering from conditions such as stroke, paralysis, traumatic brain injury, and multiple sclerosis. These advanced devices support and enhance physical movement, making them invaluable during therapy sessions. Exoskeleton robots significantly improve the rehabilitation experience while promoting user motivation and independence in daily activities by increasing the range of motion and facilitating strength-building exercises. These advanced technologies support recovery by enhancing mobility and promoting independence for individuals with these conditions. Rehabilitation is restoring patients’ physical abilities to the average level by studying their posture and gait. The World Health Organization states that 1 billion people worldwide have disabilities, with 110 to 190 million experiencing significant functional challenges. Many disabled people need rehabilitation [4]. According to the Rehabilitation Association of Uzbekistan, each year, approximately 45000 people require gait rehabilitation, compared to 795000 people in the USA [5]. Only 60 % can exercise leg muscles, and 40 % need clinical care using the gait rehabilitation exoskeletons. These statistics suggest that exoskeletons for individual patients and rehabilitation centres are essential.
This paper thoroughly examines the different classifications of exoskeletons, emphasising their design and functionality. Additionally, it explores a variety of lower limb exoskeletons specifically developed for gait rehabilitation, highlighting their roles in improving mobility and recovery for individuals with walking impairments. It studies gait anatomy, mechanical design, and control strategies and concludes with future research directions focused on the mechanical design of exoskeletons, including actuators, sensors, and control strategies.
The paper is structured as follows: the section on Methodology describes the literature-searching methodology; the section on classification presents types of exoskeletons, mainly categorised based on the lower body for gait rehabilitation. The subsequent sections will examine the introduction of lower limb exoskeletons, analyse studies pertaining to gait anatomy, outline the mechanical design, and explore the control strategies utilised for the prototype of lower limb exoskeletons. In conclusion, remarks related to actuators, sensors, and the control strategy of exoskeletons will be presented for future research.
2. Research and selecting methodology
We comprehensively reviewed research papers on lower-limb exoskeletons, sourcing materials from PubMed, MDPI, IEEE Xplore, ScienceDirect, SpringerLink, and Scopus. Our selection included articles published since January 2010. About 1236 articles were selected by using keywords: “medical robotics” and “exoskeletons”. All articles contained in our review are in English. After skimming the title and abstract, we classified the collected papers according to the adopted exoskeletons for gait rehabilitation and lower limb exoskeletons. We evaluated various lower limb fixed exoskeletons designed for gait rehabilitation, focusing on their types, designs, control strategies, and distinguishing features. We are pleased to present a prototype of a fixed lower limb exoskeleton meticulously designed to assist individuals in the process of gait rehabilitation. This innovative device holds the potential to significantly improve mobility and provide critical support for patients as they work towards regaining their walking abilities. This exoskeleton is intended to facilitate an effective recovery process and enhance overall movement efficiency through targeted therapeutic interventions and real-time feedback.
3. Classification of exoskeletons for rehabilitation
The development of medicine and the ageing of diseases are directly causing the diversification of medical devices [6]. From this point of view, we carried out scientific studies on various medical exoskeletons for different purposes. This aims to differentiate and target specific categories of exoskeletons for further research.
Currently, more than 200 exoskeletons [7] are classified according to the purpose of use, the principle of operation, and other factors. Based on the area, exoskeletons can be divided into medical, industrial, military, and consumer [8]. Industrial and military exoskeletons enhance the ability to carry heavy loads with minimal effort by boosting speed, strength, and endurance. Consumer exoskeletons help humans to level up workouts. Medical exoskeletons mainly consist of rehabilitative and assistive exoskeletons, which provide directed movement and reduce the burden on the user (rehabilitation) or relieve labour intensity by physically supporting activities of daily living (assistance). The development of exoskeletons in the military and medical fields is very high, but their practical implementation and commercialisation have not yet been fully formed.
Medical exoskeletons designed for rehabilitation serve specific purposes and can be categorised into three main types. First, body weight support exoskeletons help reduce the strain on patients by providing necessary support, allowing them to engage in rehabilitation activities with greater ease. Second, gait-assisted exoskeletons are engineered to aid individuals in walking by offering assistance with limb movement and stability, facilitating a more natural gait. Lastly, intelligently powered exoskeletons incorporate advanced technology to adapt to the user’s movements and needs, providing personalised support for rehabilitation and enhancing functional mobility. Each type contributes uniquely to the overall process of patient recovery and mobility enhancement, playing a vital role in helping individuals regain their strength and independence during the healing journey [9].
Fig. 1Three types of medical exoskeletons
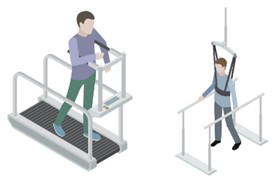
a) The body weight helps support exoskeletons
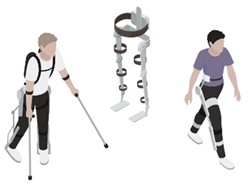
b) Exoskeletons are designed to assist individuals with walking
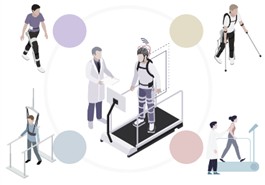
c) Advanced intelligent exoskeleton systems aimed at augmenting human performance and capabilities
Body Weight Support (BWS) Exoskeletons (Fig. 1(a)) restore patient mobility while reducing human body weight. BWS delivers advanced power-assisted support designed specifically for individuals with limited motor functions. This service aims to enhance mobility and improve the overall quality of life for patients. It is fixed and stationary. Gait-assisted exoskeletons (see Fig. 1(b)) help patients achieve voluntary movement and support intentional movement exercises. This type of exoskeleton can be both mobile and fixed. Intelligent power exoskeletons (Fig. 1(c)) use advanced technologies like AI, IoT, VR, and AR to enable intelligent and flexible adjustments, enhancing patient-exoskeleton interactions and personalising rehabilitation treatments. Intelligent powered exoskeletons are entirely mobile and soft. Such exoskeletons are relative, and it is also possible to apply modern technologies to exoskeletons of a modified form or to combine all kinds of exoskeletons. Therefore, the effectiveness and scope of the application of exoskeletons will be expanded, and productivity will increase.
On the other hand, rehabilitation exoskeletons can be divided into two main categories: partial body movement or independent walking after rehabilitation [10]. The partial-body exoskeletons were designed to enhance locomotion by providing additional torque and power to users. The independent walking exoskeletons are more advanced, portable, commercial devices like Rewalk and Ekso [10]. That classification is more specific, but rehabilitation exoskeletons are divided into three types based on the mechanical design and body parts: ground exoskeletons, end-effector tools, and wearable exoskeletons [11-13]. Ground exoskeletons and the treadmill should be fixed. Medical exoskeletons for ground (fixed frame) should be assistive and rehabilitative. Each model has at least two actuated joints: the hip and knee, or sometimes just one, with the ankle controlled. Fixed exoskeletons for gait rehabilitation are bilateral devices, and their primary involvement requires upper limb aids to maintain balance. In addition to the devices, they provide leg movement and body weight support (BWS) and ensure safety. The end-effector concept outlines the positioning of the patient's foot on the footboards, facilitating the turning steps involved in the gait retraining process. This includes programmed motors or passive components that control the movement of the knees and hips during walking. Wearable exoskeletons [14-16] are rapidly growing as a breakthrough gait retraining technology due to their ability to encourage active client participation in exercise and be used as assistive devices for the public. Following the current trend towards robotic devices, research into wearing exoskeletons has increased over the past 10 years.
The primary forms of rehabilitation exoskeleton used are for patients with limited lower limb mobility, and the wearable form is now widely developed. Scientific research on the ground form is somewhat limited due to the lack of public demand and the clinical nature of the requirements.
Exoskeletons for rehabilitation can be classified into three categories based on their use of power: passive exoskeletons, quasi-passive exoskeletons, and powered exoskeletons, which are considered active forms [17-18]. Passive exoskeletons convert part of human energy into mechanical energy, heat energy, friction energy, and vibration energy by controlling the joint movement of the human body using people’s metabolic energy [19] and elastic energy storage mechanisms. According to the energy conservation law, some energy that should be used is recycled into the system. This process reduces the metabolic energy within the system, which helps promote movement in the human body and improves energy utilisation. A passive element and support generate a moment when a small actuator is connected or disconnected by quasi-passive exoskeletons, forming a quasi-passive device. The device is referred to as a Clutch Elastic Actuator (CEA). Users can achieve seamless mobility in unassisted tasks with passive assistance, enabling exoskeletons to engage or disengage as needed. Powered exoskeletons serve as energy sources that feed electric, pneumatic, hydraulic, and other forms of energy into the human energy system. According to the energy conservation law, additional energy supplied to the body reduces the initial metabolic energy needed, decreasing overall energy consumption.
Fig. 2 presents the types of exoskeletons divided for rehabilitation based on functionality, elements, power source, and body parts.
Functional classification of exoskeletons here is an attempt to define the type of device suitable for a particular application.
Fig. 2Classification of exoskeleton robots
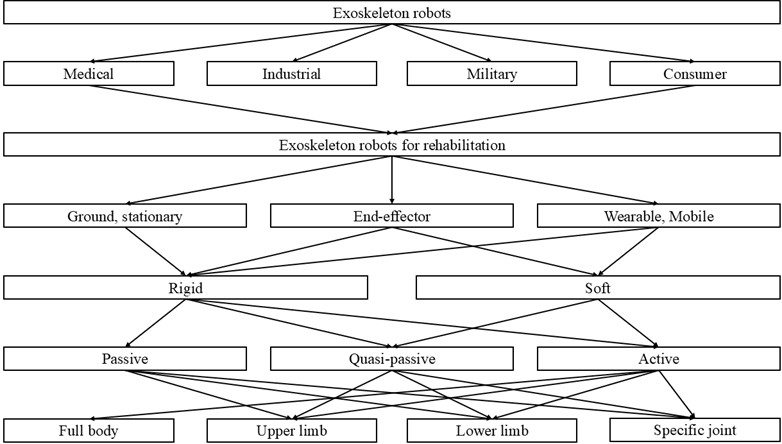
4. Applications of lower limb exoskeletons in gait rehabilitation
Exoskeletons are categorised into four types (Fig. 3) according to the body parts they have been designed: exoskeletons for the entire body, upper body, lower body, and specific segments [17, 20-21]. Full-body exoskeletons are advanced devices specifically engineered to offer extensive support for the entire body. These innovative systems enhance mobility by allowing individuals to move more freely and easily. By distributing weight and reducing strain on muscles and joints, full-body exoskeletons significantly improve functionality, making everyday tasks more accessible for those with mobility challenges. In general, they will have about 50 DOFs. Such devices are more complex and primarily mobile.
Fig. 3Lower limb exoskeletons intended for gait rehabilitation may incur significantly higher expenses
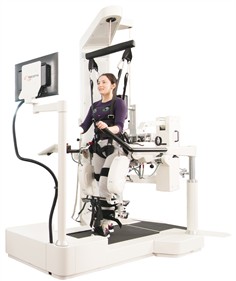
a) Lokomat exoskeleton [3, 5]
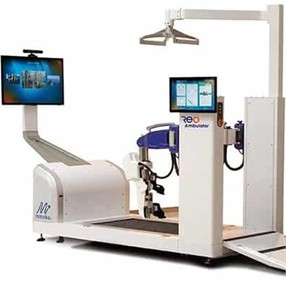
b) ReoAmbulator exoskeleton [7]
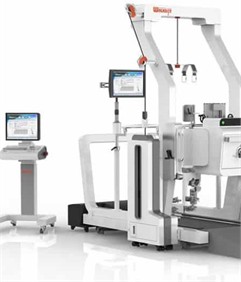
c) Walkbot exoskeleton [7]
The lower limb fixed exoskeleton for gait rehabilitation is an essential category of rehabilitation robots designed to assist individuals in restoring walking functions. This device is anchored to the body, ensuring stability and support while facilitating controlled movements of the lower body joints, such as the hips, knees, and ankles. During rehabilitation sessions, the exoskeleton actively guides the user’s movements, retraining gait patterns and muscle activity. By providing this targeted support, the exoskeleton helps individuals strengthen their lower limbs, improve balance, and regain confidence in their mobility, ultimately enhancing their overall rehabilitation experience. Research and technological work on lower limb fixed exoskeletons began in the 1960s [22]. In 1970, the first active exoskeleton for the lower body was developed, featuring three degrees of freedom per leg [23]. It was named Belgrade Exoskeleton [24] for gait assistance. It was designed to assist individuals with neurological conditions, such as those resulting from stroke or spinal cord injury (SCI) [25]. Research activity on exoskeletons increased in 2017, and exoskeletons became popularised in the health market after regulatory approval [26]. In the last 14 years, scientists have presented various designs of exoskeletons in this area. A renewed prototype and interest in these old designs are now leading to a cutting-edge scientific and technological aspect attracting research teams.
The lower limb rehabilitation exoskeleton consists of a one-to-one connection between the exoskeleton robot and human joints, and a preprogrammed trajectory guide supports every single joint. Lower limb exoskeletons equipped with fixed frames are specifically designed to provide assistance and rehabilitation for users. These sophisticated devices enhance mobility by facilitating movement while promoting recovery and strength development in the lower limbs. They typically have at least two actuated joints, the hip and [knee, while the ankle is usually controlled. Fixed exoskeletons for gait rehabilitation are bilateral devices, and the central involvement requires upper limb aids to maintain balance [27]. In addition to the devices, they provide leg movement and body weight support (BWS) and ensure safety [28].
The lower limb fixed exoskeleton for gait rehabilitation is designed to assist patients in simulating standard walking patterns while effectively strengthening their leg muscles. This innovative device incorporates mechanisms that replicate human footsteps, facilitate precise posture control, and provide a supportive body weight system, enhancing users' rehabilitation experience [29].
In the given examples (Table 1), five exoskeletons that compose the group named “lower body fixed rehabilitation” are fully explained based on the following features [4, 31]: the applied segment (lower body area: hip, knee, ankle), the number of DOF, the type of actuators (electric, hydraulic, and pneumatic), the powered system (active, passive, quasi-passive), the types of sensors (physical, bio-signal, environmental), control system (position, force, impendence, EMG based, adaptive control).
Table 1Commercial lower limb fixed exoskeletons
Exoskeletons | Company, country | Body area | DOF | Control system | Powered system | Actuators | Sensors |
Lokomat [3, 5, 6, 9, 28, 29- 32, 38] | Hokoma AG, Switzerland | Hip-Knee | 2 | Impedance control | Active | DC motor | Physical and biosignal sensors |
ReoAmbulator [3, 29, 33, 34, 35] | Motorika, USA | Hip-Knee | 2 | Adaptive control | Active | Electric | Physical and biosignal sensors |
Walkbot [6, 13, 30, 36-39] | P&S Mechatronics, South Korea | Hip-knee-ankle | 3 | Adaptive | Active | AC-DC Servo motors | Physical signal sensors |
MRG-P110 [40, 41] | “HIWIN” Robotic Gait Training System, Taiwan | Hip-knee-ankle | 2 | Impedance control | Active | Electric | Physical signal sensors |
Gait simulator | Center Rehabilitation, Russia | Hip-knee-ankle | 2 | Force control | Passive | No | No |
4.1. Lokomat
The Lokomat, developed by Hocoma AG in Switzerland, is a bilateral gait rehabilitation robot with a body weight support (BWS) system. The patient's legs are moved in the sagittal plane using DC motors connected to ball screws. The hip and knee joints of the Lokomat are powered by engines with linear ball screws [28]. In contrast to the Lokomat, the latest generation of exoskeletons is designed to focus on low mechanical impedance. The Lokomat is equipped with cutting-edge active actuators located at the hip and knee joints, which work in concert to guide a patient’s limbs expertly. These sophisticated devices enable the Lokomat to ensure precise movement in the sagittal plane, meticulously following a predetermined trajectory designed for effective rehabilitation. This technology allows patients to experience tailored guidance that promotes proper gait patterns and enhances their recovery journey. Furthermore, the Lokomat features an impedance controller that modifies the “guidance level”, which defines the permissible degree of deviation from the intended limb movement pattern. This integration guarantees optimal support and rehabilitation for patients [9].
Woodway has developed KineAssist and Lokohelp in the U.S., while Maibu Robotics in China has created the MoonWalker. These innovative exoskeleton robots are engineered to lighten users' load while improving their running efficiency significantly. By utilising advanced materials and technology, these devices support the wearer’s movements, allowing for more incredible speed, less fatigue, and enhanced performance during physical activities. The MoonWalker includes customisable movement modules, adjustable intensity levels, visual control options, and real-time feedback to improve user experience and adaptability [9].
The updated version of Lokomat features an optional FreeD module that enhances therapy by enabling pelvic lateral translation and transverse rotation [28].
The Lokomat is the first gait orthosis designed to assist individuals with walking difficulties using a treadmill. It coordinates the movement of the hip and knee joints, and for the ankle joint, it employs an ankle-foot orthosis equipped with a plantar flexion stop spring [30].
The Lokomat’s trajectories can be customised to suit individual patients and their specific step lengths [28]. It is one of the more well-researched stationary robotic systems developed to support and automate treadmill training [31].
4.2. ReoAmbulator
ReoAmbulator, developed by Motorika Ltd. and marketed in the USA as the “AutoAmbulator”, is a robotic system that supports body weight during treadmill use [3]. Robotic arms are strapped to the patient’s legs at the thigh and ankle, driving them through a stepping pattern. A single-masked, randomised clinical trial is underway to assess the effectiveness of ReoAmbulator in stroke patients. ReoAmbulator was developed in collaboration with the HealthSouth network of rehabilitation hospitals [29].
The ReoAmbulator robotic system, also referred to as “AutoAmbulator”, is an example of a treadmill gait trainer designed for lower-limb rehabilitation therapy, as illustrated in Fig. 2(b). The ReoAmbulator system is widely used in research centres and medical hospitals for rehabilitation therapies and educational studies on motor function recovery. Central to this system is a powered leg orthosis with advanced robotic arms that help patients engage in gait motion while providing additional walking force, enhancing rehabilitation. The robotic arms are attached to the thigh and ankle, allowing for a controlled stepping pattern tailored to each patient’s needs. This technology not only aids physical movement but also promotes neurological adaptation for better locomotion. Studies show that robot-assisted gait training can significantly improve balance and gait, comparable to traditional manual therapies. The ReoAmbulator stands out among commercial rehabilitation orthoses due to its significant development and the introduction of various prototypes that enhance its effectiveness in diverse rehabilitation settings [33].
Multiple sensors continuously monitor and adjust power and speed according to the patient’s needs. An interactive display with various modes boosts motivation and adds variety to training exercises. The ReoAmbulator’s sensors can assess mobility before and after training, allowing Motorika to customise experiences and track progress. The exoskeleton is connected to a virtual reality simulator featuring diverse terrains and games to enhance engagement. It also provides verbal and audio feedback. One mode displays an ideal step pattern for the user to replicate, with adjustments possible on the spot. A side panel enables a professional to control the system directly. The ReoAmbulator is a specialised device intended for individuals engaged in gait rehabilitation. Its primary objective is to enhance various aspects of locomotion, including gait patterns, balance, weight-bearing, coordination, speed, and endurance during walking activities. This innovative tool supports rehabilitation by providing targeted assistance and promoting functional improvement [34]. This system operates in a tethered manner akin to the Lokomat [35].
4.3. Walkbot
The Walkbot is an advanced fixed-frame exoskeleton designed explicitly for gait rehabilitation. It is offered in three distinct models to accommodate varying needs: Walkbot_S (standard model), Walkbot_K (pediatric model), and Walkbot_G (next-generation model) [36]. The Walkbot series of gait rehabilitation exoskeletons is an innovative advancement by the South Korean company P&S Mechatronics. Notably, two of the three models are designed for pediatric use through their inherent design or by incorporating additional modules. The Walkbot stands out from other fixed-frame gait rehabilitation exoskeletons due to its powered hip-knee-ankle design, which enhances its functionality. It is an effective evaluation device and features the Walkbot_G model, equipped with an integrated pressure plate to monitor each step in real-time. Furthermore, the device’s full integration with virtual reality provides an engaging platform for interactive training, demonstrating its commitment to improving rehabilitation outcomes.
The Walkbot system is an innovative gait orthosis designed to precisely coordinate hip, knee, and ankle movements through advanced natural gait training techniques. It addresses foot drop and toe drag by controlling ankle dorsiflexion and plantar flexion [30].
The device is equipped with a suspension harness designed to provide body weight support (BWS), a motorised treadmill, and an actuator-controlled exoskeleton (Fig. 3(c)). The treadmill’s speed and torque can be adjusted to provide both assistance and resistance, allowing for customisation according to the patient’s level of locomotor performance as training progresses [37].
4.4. MRG-P110
The “HIWIN” Robotic Gait Training System, specifically the model MRG-P110, is an advanced, fixed-frame medical robot tailored for gait rehabilitation. Unlike traditional rehabilitation methods, this innovative device remains stationary, allowing patients to benefit from its support while their legs are gently guided through a walking motion. This unique approach enables the rest of the patient’s body to remain stable, minimising unnecessary movement.
The MRG-P110 facilitates rehabilitation by assisting users in effectively retraining their walking gait cycle. It operates like a sophisticated powered leg exoskeleton firmly anchored to the ground. As the patient “walks” across specially designed actuated plates, the exoskeleton provides the necessary power and guidance to promote proper leg movement.
Moreover, this state-of-the-art device is fully integrated with virtual reality technology, creating an interactive and engaging training environment. This integration enhances user motivation and provides valuable feedback to optimise the rehabilitation experience, helping individuals regain mobility and confidence in walking [40].
Fig. 4Lower limb exoskeletons are designed for gait rehabilitation at a more accessible cost
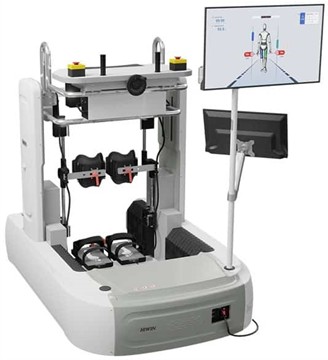
a) MRG-P110 [7]
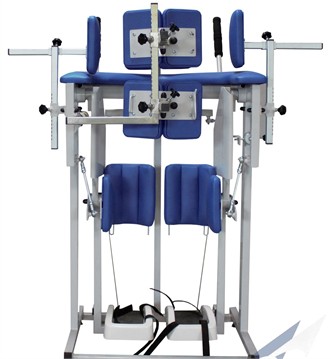
b) Gait simulator
4.5. Gait simulator
The Gait Simulator is an innovative rehabilitation device specifically crafted to restore and enhance motor skills in the lower extremities, primarily in Russia’s medical care context. This advanced simulator plays a crucial role in the recovery process for patients who have experienced conditions affecting the brain and spinal cord.
The use of this specialised medical simulator is particularly beneficial for individuals dealing with various challenges, including paresis (weakness), paralysis (loss of movement), and muscle contractures (shortening of muscles). It is also indicated for patients suffering from the lasting effects of cerebral palsy, various neurological disorders, and any conditions that lead to compromised mobility or heightened spasticity in the legs.
The unique design of the Gait Simulator allows patients to initiate motion through their arms. Users can synchronise the movement of all simulator components by moving their arms (or even just one arm), leading to the involuntary movement of their otherwise stationary lower limbs. For patients who have sustained injuries to the cervical and upper thoracic regions of the spinal cord, additional supportive apparatus is available to ensure safety during rehabilitation.
In these cases, patients are carefully positioned upright, and this support enables them to participate in a comprehensive range of rehabilitation exercises, all aimed at fostering strength, coordination, and improved mobility in their lower extremities. The Gait Simulator thus offers a holistic approach to rehabilitation, empowering patients on their journey to recovery.
In the given examples (Table 1), five exoskeletons that compose the “lower body fixed rehabilitation” group are fully explained based on their features; only one is passive and is under our subsequent research.
5. Prospective initiatives in research and development activities
Our long-term outcome is that a modernised prototype of the gait simulator will become a lower-cost and smarter gait rehabilitation exoskeleton that stroke patients can use at their home setting and clinical centre. An exoskeleton intended for gait rehabilitation is a sophisticated wearable robotic device that aids in the recovery and enhancement of walking abilities. It is modernised based on artificial intelligence and mechanical power plants, which include modern control technology, computer technology, sensor technology, and intelligent robot technology [42]. In the past few years, exoskeleton developers have tried to develop its performance, wearability, and portability [43]. We will integrate state-of-the-art technology mechanics, sensor technology, control systems, and artificial intelligence into developing our Gait Simulator prototype. This comprehensive approach aims to enhance the simulator’s functionality and performance.
The new prototype of the Gait Simulator will be researched and developed based on the following aspects.
5.1. Gait anatomy for the prototype
The lower limb gait system primarily comprises a network of bones and joints, as illustrated in Fig. 5. There are seven types of bones, nearly 300 muscles, and three joints: hip (provided 3 DOFs), knee (provided 1 DOF), and ankle (provided 3 DOFs) [44-46]. Lower body movements are categorised into three primary groups: flexion and extension, adduction and abduction, and internal and external rotation. This classification provides a clear framework for understanding the functional mechanics of lower body dynamics [4], [47-48].
Fig. 5The joints and bones of the lower body
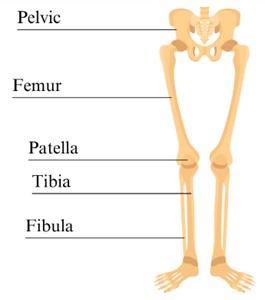
Fig. 6The sagittal plane of the lower body
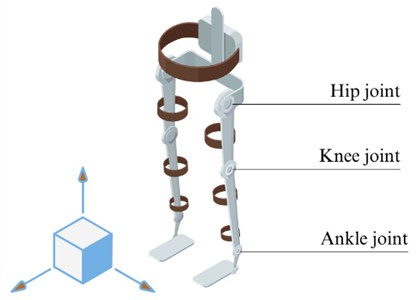
Lower body exoskeletons can be targeted at single-joint or multi-joint levels. While the single joint may be applied to one specific joint, the multi-joint may involve a combination of lower joints [49].
To make the gait rehabilitation exoskeleton have similar movement functions to the lower body, some researchers sometimes select the 15 DOFs, but this kind of design makes the system more complex [50]. Our research focused on this situation in the sagittal plane based on Table 2 as follows as a multi-joint:
1) Hip joint connected to pelvic and femur bone, allowing flexion/extension rotation [51].
2) The knee joint contains the patellofemoral joints, and its movements support the flexion/extension during walking [51].
3) The movements of the ankle joint primarily occur in the talocrural joint, allowing for plantar flexion and dorsiflexion [28], [51-52].
The gait patterns observed in patients exhibit a high degree of complexity arising from their compromised motor functions. However, we can characterise the gait process via step length, width, and speed parameters. Our studies focused on the effects of various parameters on joint angles and their relationship with gait parameters. Reference [48] offers an in-depth exploration of the essential movements involved in human walking gait, providing a detailed examination of each phase and the intricacies of how they contribute to efficient locomotion. This analysis highlights the dynamic interplay of various muscle groups and joint mechanics that facilitate smooth and coordinated walking patterns. The range of motion has a significant role in exoskeletons. In [4], the range of motion is discussed and represented in the table of human lower extremity ranges, including the joints, movements, and values of rotate angels, compared between the sources: American Agency of Orthopedic Surgeons (1965), American Medical Association.
Table 2DOFs, bones, and joint movements of the lower body in the sagittal plane
Joints | Bones | DOF | Joint movements | Movement activities | Examples |
Hip joint | Femur | 1 | Flexion-extension | ![]() ![]() | Honda Walking, Assist, HiBSO, PH-EXOS, Exosuit |
Knee joint | Tibia, patella | 1 | Flexion-extension | ![]() ![]() | Knee Exo/Carnegie Mellon University, EXO |
Ankle joint | Fibula | 1 | Dorsiflexion-plantar flexion | ![]() ![]() | MIT ankle exoskeleton, BIT Ankle, Knee soft exosuit |
5.2. Mechanical design for the prototype
Mechanical design plays a vital role in the functionality of lower limb rehabilitation exoskeletons, as it ensures the efficient transmission of force and energy through its integration with the human lower body. The anthropometric dimensions are also a significant part of the design concept [53-54]. These objectives can be accomplished by carefully designing suitable robotic mechanisms and actuation systems. [28]. In the design process, we must consider motion trajectory design, the comfort of human-machine interaction, and the effectiveness of rehabilitation activities [55-56].
Fig. 7 shows the renewed prototype of exoskeletons, according to the anatomy structure of the lower body explained in Section 5.1. Electric motors drive the prototype's mechanical design.
Fig. 7Mechanical design of the prototype
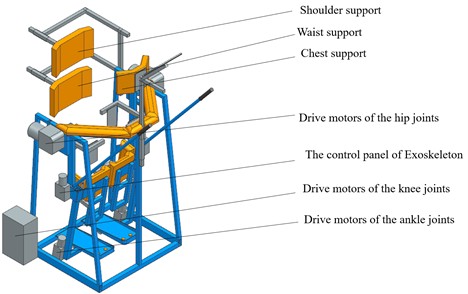
The kinematic structure of exoskeletons is the central part of mechanical design and is distinguished depending on the category of its constituent elements. It has two types [20].
Soft exoskeletons. Soft exoskeletons consist of flexible and lightweight elements that aid human movement. They are based on passive-assistive control and are wearable, mobile, and portable [76].
Rigid exoskeletons. The rigid exoskeleton consists of actuators, joints, and kinematic joints that enable and enhance human movement. In the medical field, two distinct types of exoskeletons are commonly employed, with specific designs incorporating features from both types for improved functionality.
5.3. Control structure of the prototype
The design of lower limb exoskeletons involves several essential components that work together to enhance mobility and support for the user. These components include advanced sensing systems that detect movements and provide real-time feedback, powerful actuation mechanisms that generate the necessary forces for movement, and sophisticated control strategies that ensure seamless coordination and responsiveness between the exoskeleton and the user’s intentions. Together, these elements create a functional and efficient device capable of improving mobility and aiding rehabilitation. These elements are detailed in references [46] and [56-57]. The control strategy is mainly due to the complex interaction between humans and robots and the human-robot interaction’s cognitive and physical aspects [58].
The control strategy for lower limb exoskeletons encompasses several critical components, including defined gait patterns, specific training objectives, a standardised evaluation framework for rehabilitation, and relevant motion data. Exoskeleton control strategies used in gait rehabilitation can be broadly categorised into two primary types. These categories encompass various approaches designed to enhance mobility and improve walking patterns for individuals undergoing therapy: (1) trajectory tracking and (2) assistance as needed. This classification provides a clear framework for understanding the approaches utilised to enhance gait recovery. In trajectory tracking, gait pattern activities were preprogrammed, like a normal gait. Gait patterns include gait time, interjoin coordination, and hip, knee, and ankle angles. The primary purpose of rehabilitation gait training is to restore the functions of disabled patients to normal levels in the lower body.
The most prevalent methodologies employed in the control of exoskeletons are force-based control and position-based control. These approaches are widely recognised for their effectiveness in enhancing the functionality of exoskeletal systems [59]. The specific control systems for exoskeletons should be selected individually or combined; however, a combined approach complicates the evaluation of the system. In the development of rehabilitation, exoskeletons are usually controlled position-based; in some resources, it is discussed as a “gait trajectory control” [60-61]. This control system is based on desired prerecorded exoskeleton positions (e.g., joint angles).
Position control and trajectory tracking guide the patient's lower limbs by providing feedback that aligns with fixed reference walking trajectories based on joint angles. It includes an internal control loop using the error between the reference angle trajectories and the angles measured by the sensors at each lower limb joint. A positive point of this control strategy is the imposition of a predefined joint angle trajectory resulting in limited kinematic error, an influential factor in driving human motor learning [75].
Torque control enhances versatility, robust behaviour, and dependable and safe tasks in the presence of humans. Therefore, torque control is widely employed in exoskeletons. This control method uses the error between the reference torques and measured torques by sensors on each actuator. Since torque control closely interacts with actuators, it delivers a simple way of controlling energy flow from the exoskeleton to the user, which is helpful in biomechanics research [75].
Fig. 8The Human-exoskeleton collaboration system
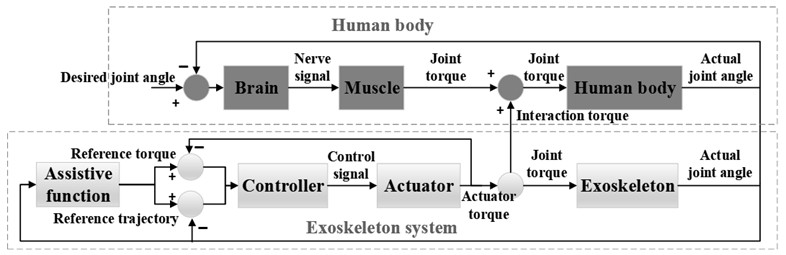
Control system design has been developed in the last decade, but there are still some dissolved problems related to human-robot integration in control systems [28], [62]. One of the largest is whether the patient should organically combine with the robot. The solution appears to integrate the human body and robot properly.
Moreover, the torque control method is critical in implementing the rigid-body inverse-dynamics control strategy. Although position control is common in robotics, it is not appropriate for all tasks, particularly when robots need to interact physically with the environment.
5.4. Actuators for the prototype
Currently, electrical motors are used wider than hydraulic and pneumatic types [63-64], more discussed in [59-60], [65].
The pneumatic actuator operates using a pressurised air source, which can be advantageous; however, it may have some limitations, such as lower power output and potential challenges in control. The principle of operation of pneumatic actuators is like that of hydraulic actuators, but it uses compressed inert gases instead of liquids. It also allows compressed air from the tank to increase the pressure inside the piston or diaphragm, causing it to expand or reduce the pressure at the outlet and cause it to contract [73].
Hydraulic actuators are typically characterised by their substantial size and higher cost, which can be attributed to their significant power requirements and the necessity for stability [48]. They are mainly used in Exoskeletons, designed to increase the user's strength in industrial or military applications. The principal advantage of a hydraulic actuator resides in its capacity to generate substantial power output. Still, on the other hand, the complexity of working with high-power liquid pumps and the weight of the liquid pumps and pipes are its disadvantages [73-74].
Being controllable of electric actuators is more suitable than others. The most used electrical motors are servo motors capable of advanced position-based control and can control many degrees of freedom, from 1 to 22. There are also other types of electric motors: AC motors, Brush DC Motors, and Stepper motors [4]. DC motors are well-known for their high torque-to-weight ratio, low noise, and reliability. One of the more feasible electric motors is a battery used to power electric actuators [44], [59]. Electric actuators will lead to the development of modern exoskeletons, as their parameters are size, weight, torque, and velocity. In developing lower limb exoskeletons, it is recommended to prioritise the placement of actuators on a single joint. This approach can enhance functionality and efficiency in the overall design. This approach facilitates the simultaneous control of multiple joints and allows for coordinated movement across two or more joints. This approach can enhance the overall functionality and effectiveness of the exoskeleton system. The Series Elastic Actuator (SEA) drive marks a considerable technological advancement, characterised by its ability to enhance performance by integrating elastic elements. This design improves precision and compliance in motion control applications, making it an asset in various engineering fields. It provides exceptional control precision, enhances safety, mitigates inertia impact, and improves energy efficiency by reducing friction losses. This innovative solution is poised to meet high-performance standards in various applications [66].
Actuator-related forms of exoskeletons can use single-class actuators or different-class actuators. However, in practice, one-class actuators are mainly used. Although using multi-class actuators is theoretically correct, it is more complicated.
Table 3Actuators for exoskeletons.
Actuator type | Advantages | Disadvantages |
Pneumatic | - Low cost - Clean and fast air system - Safety | - Limited power - Difficult control - Maintenance and Leakage Issues |
Hydraulic | - More genuine - Dissipating heat - More stable - Easy to control | - Cumbersome - High-cost - Low drive speed - Low energy efficiency |
Servo motor | - Simple control - Exhibits exceptional movement precision - Quick response - Minimum price - Simple structure | - The influence of external charges on stability is minimal - More inertia - Weightiness |
SEA drive | - High accuracy in control force - Enhanced security with minimised inertia impact - Maintaining a stable human-machine connection | - Elastic tools limit rigidity - Huge volume - Hefty weight - Complex design - Huge power |
5.5. Sensors for the prototype
In the past decade, sensors have become the central part of the exoskeletons, making them more attractive and safer. According to the types of signals in medical exoskeletons, mechanical sensors help to control the position, altitude, force, torque, pressure, and acceleration based on mechanical signals (or physical signals) are measured by potentiometers, piezoelectric sensors, capacitive sensors, accelerometers, gyroscopes, a foot sensitive resistor [44], etc. Neural and brain signals (or biosignals) need to sense human body elements during the rehabilitation training [67] to analyse the patient’s recovery and plan the following training proposals. Some offered sensors for measuring the bio-signals: EMG sensors [44], EEG, fNIRS, and MMG [8].
In addition, it has a positive effect on the efficiency of exoskeletons. Exoskeletons can be categorised into various types depending on their specific sensor environments. These categories reflect the different ways in which these advanced devices interact with their surroundings and respond to external stimuli [8], [10].
Exoskeletons directly to the physical sensing system. The inertia, force, and displacement sensors control the exoskeleton and measure kinematic parameters. They help track the exoskeleton’s positions, movements, forces, and the user’s body. Inertial sensors measure the orientations, positions, and movements of exoskeletons and patients using accelerometers, gyroscopes, and magnetometers, often combined into IMUs. Force sensors are employed to accurately measure the forces and torques exerted on joints, which facilitates a comprehensive evaluation of human-robot interactions. The exoskeletons can contribute to the system’s accuracy and control using the displacement sensors.
Fig. 9Sensor’s cooperation
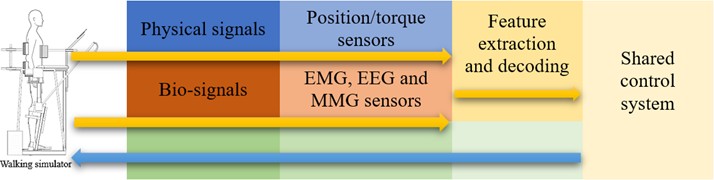
Environmental sensors for exoskeletons are essential in improving their interaction with the environment. Various sensors, including ultrasonic, infrared, and LiDAR, can seamlessly integrate these advanced mobility aids. These sensors facilitate critical functions such as detecting real-time obstacles, avoiding potential collisions, and enabling smooth navigation through diverse terrains. By providing essential data about the exoskeleton's surroundings, these sensors significantly improve safety measures and increase adaptability, allowing users to manoeuvre confidently in different environments, whether indoors or outdoors. Exoskeletons equipped with human signal monitoring can control biological signals, enabling the analysis and determination of the patient’s physiological state. These devices primarily utilise sensors such as EMG (electromyography), MMG (mechanomyography), and EEG (electroencephalogram) to offer valuable insights into the patient’s physical condition, levels of exertion, and cognitive processes.
In general, incorporating the three types of sensors into exoskeletons will make them more expensive and advanced but also shorten, more effectively, and safer the patient’s treatment process.
6. Conclusions
This research explored five distinct robotic systems specifically engineered as fixed exoskeletons to facilitate the rehabilitation of the lower limbs. Each innovative device aims to enhance mobility and support recovery for individuals with mobility impairments, providing a structured approach to therapy and rehabilitation. Each system was assessed for its effectiveness in improving mobility and recovery for individuals with gait impairments. The selected fixed exoskeletons are more complex mechatronic devices than other types. Many research papers on lower limb exoskeletons for the laboratory were found, but the commercial type of exoskeletons is much less discussed in detail. This publication reviewed five types of commercially available lower limb fixed exoskeletons by developing a comparison table. The discussion surrounding lower limb exoskeletons has been expanded, and we are prepared to present our upcoming initiatives. The classification table was created based on selected items. In the next section, the gait anatomy of lower limb exoskeletons, control strategy including actuators and sensors, and mechanical design for the prototype were studied and discussed for future research activities.
Developing the prototype of the exoskeleton's following factors is more critical.
Energy efficiency. In the future, energy technology will be a significant part of exoskeletons, using solar energy, bioenergy, nanotechnology, and wireless charging systems [65]. Improving the energy efficiency of exoskeletons is essential for extending their operational duration. Exploring innovative structures capable of effectively storing and releasing energy during the various phases of the gait cycle is recommended. This approach could significantly enhance the performance and functionality of exoskeleton systems [43].
The physical stops should be meticulously designed to withstand the maximum torque produced by the actuators reliably. This ensures optimal performance and safety in operation. Safety measures must be incorporated into the exoskeleton’s control system. Furthermore, additional control strategies must be developed to ensure the wearer's stability and safety in emergency situations [43].
Human-Robot Collaboration. The research team has proposed a new concept called “human-in-the-loop,” in which the human and robot are integrated into a closed loop, and the robot can adjust the working conditions according to the human’s walking condition. This type of human-robot co-fusion could be a trend for developing rehabilitation robots [65].
Lower costs. The expense associated with developing lower limb exoskeletons poses a significant challenge. Currently, the available exoskeleton systems are prohibitively expensive for most individuals with mobility disorders, ranging from $100,000 to $130,000. These amounts create significant affordability challenges for many people needing such technology. Researchers should focus on creating exoskeletons accessible to a more substantial number of disabled individuals. Recent advancements in robotics can potentially significantly lower the costs associated with high-performance actuators and sensors. This reduction of expenses could enhance the affordability of exoskeleton systems, making them more accessible for a broader range of applications. Financial limitations will likely influence patients' choices and willingness to use exoskeletons. Thus, enhancing the design and lowering costs will facilitate greater patient acceptance and utilisation of exoskeletons for gait rehabilitation [68].
Lower weight. The exoskeleton must support the user's weight and his weight [69]. There are some methods to reduce the device’s weight related to the actuators and materials of exoskeletons. The system comprises a blend of active actuators, which actively control movement and respond to input signals, and passive actuators, which rely on external forces to operate without requiring direct control. This combination enhances functionality and efficiency in various applications [48]. It can help because passive actuators are more lightweight than active ones, but the results are not at a high level. Selecting light and materials is a more effective method [64]. Applying carbon fiber composites can effectively reduce the overall weight of exoskeletons, enhancing their performance and usability. Furthermore, utilising three-dimensional printing technology to produce specific components can produce highly favourable manufacturing results [43].
References
-
A. A. Morgan, J. Abdi, M. A. Q. Syed, G. E. Kohen, P. Barlow, and M. P. Vizcaychipi, “Robots in healthcare: a scoping review,” Current Robotics Reports, Vol. 3, No. 4, pp. 271–280, Oct. 2022, https://doi.org/10.1007/s43154-022-00095-4
-
S. Kar, “Robotics in healthcare,” in 2nd International Conference on Power Energy, Environment and Intelligent Control (PEEIC), pp. 78–83, Oct. 2019, https://doi.org/10.1109/peeic47157.2019.8976668
-
O. Boubaker, “Medical robotics,” in Control Theory in Biomedical Engineering, Elsevier, 2020, pp. 153–204, https://doi.org/10.1016/b978-0-12-821350-6.00007-x
-
S. K. Hasan and A. K. Dhingra, “State of the art technologies for exoskeleton human lower extremity rehabilitation robots,” Journal of Mechatronics and Robotics, Vol. 4, No. 1, pp. 211–235, Jan. 2020, https://doi.org/10.3844/jmrsp.2020.211.235
-
O. Unluhisarcikli, M. Pietrusinski, B. Weinberg, P. Bonato, and C. Mavroidis, “Design and control of a robotic lower extremity exoskeleton for gait rehabilitation,” in IEEE/RSJ International Conference on Intelligent Robots and Systems (IROS 2011), pp. 4893–4898, Sep. 2011, https://doi.org/10.1109/iros.2011.6094973
-
R. A. Gavrila Laic, M. Firouzi, R. Claeys, I. Bautmans, E. Swinnen, and D. Beckwée, “A state-of-the-art of exoskeletons in line with the WHO’s vision on healthy aging: from rehabilitation of intrinsic capacities to augmentation of functional abilities,” Sensors, Vol. 24, No. 7, p. 2230, Mar. 2024, https://doi.org/10.3390/s24072230
-
“Exoskeleton Catalog.” Exoskeleton Report, 2024, https://exoskeletonreport.com/product-category/exoskeleton-catalog/
-
S. Neťuková et al., “Lower limb exoskeleton sensors: state-of-the-art,” Sensors, Vol. 22, No. 23, p. 9091, Nov. 2022, https://doi.org/10.3390/s22239091
-
Y. He et al., “Exoskeleton-assisted rehabilitation and neuroplasticity in spinal cord injury,” World Neurosurgery, Vol. 185, pp. 45–54, May 2024, https://doi.org/10.1016/j.wneu.2024.01.167
-
Y. Yao, D. Shao, M. Tarabini, S. A. Moezi, K. Li, and P. Saccomandi, “Advancements in sensor technologies and control strategies for lower-limb rehabilitation exoskeletons: a comprehensive review,” Micromachines, Vol. 15, No. 4, p. 489, Apr. 2024, https://doi.org/10.3390/mi15040489
-
V. Warutkar, R. Dadgal, and U. R. Mangulkar, “Use of robotics in gait rehabilitation following stroke: a review,” Cureus, Vol. 14, No. 11, Nov. 2022, https://doi.org/10.7759/cureus.31075
-
G. Rodriguez Tapia, I. Doumas, T. Lejeune, and J.-G. Previnaire, “Wearable powered exoskeletons for gait training in tetraplegia: a systematic review on feasibility, safety and potential health benefits,” Acta Neurologica Belgica, Vol. 122, No. 5, pp. 1149–1162, Jul. 2022, https://doi.org/10.1007/s13760-022-02011-1
-
J. Bessler, G. B. Prange-Lasonder, R. V. Schulte, L. Schaake, E. C. Prinsen, and J. H. Buurke, “Occurrence and type of adverse events during the use of stationary gait robots-a systematic literature review,” Frontiers in Robotics and AI, Vol. 7, Nov. 2020, https://doi.org/10.3389/frobt.2020.557606
-
S. Mcdevitt et al., “Wearables for biomechanical performance optimization and risk assessment in industrial and sports applications,” Bioengineering, Vol. 9, No. 1, p. 33, Jan. 2022, https://doi.org/10.3390/bioengineering9010033
-
C. Thalman and P. Artemiadis, “A review of soft wearable robots that provide active assistance: Trends, common actuation methods, fabrication, and applications,” Wearable Technologies, Vol. 1, p. 14, Sep. 2020, https://doi.org/10.1017/wtc.2020.4
-
Y. He, D. Eguren, T. P. Luu, and J. L. Contreras-Vidal, “Risk management and regulations for lower limb medical exoskeletons: a review,” Medical Devices: Evidence and Research, Vol. 10, pp. 89–107, May 2017, https://doi.org/10.2147/mder.s107134
-
X. Tang et al., “A wearable lower limb exoskeleton: reducing the energy cost of human movement,” Micromachines, Vol. 13, No. 6, p. 900, Jun. 2022, https://doi.org/10.3390/mi13060900
-
S. K. D. Mak and D. Accoto, “Review of Current Spinal Robotic Orthoses,” Healthcare, Vol. 9, No. 1, p. 70, Jan. 2021, https://doi.org/10.3390/healthcare9010070
-
R. Baud, A. R. Manzoori, A. Ijspeert, and M. Bouri, “Review of control strategies for lower-limb exoskeletons to assist gait,” Journal of NeuroEngineering and Rehabilitation, Vol. 18, No. 1, Jul. 2021, https://doi.org/10.1186/s12984-021-00906-3
-
O. Flor-Unda, B. Casa, M. Fuentes, S. Solorzano, F. Narvaez-Espinoza, and P. Acosta-Vargas, “Exoskeletons: contribution to occupational health and safety,” Bioengineering, Vol. 10, No. 9, p. 1039, Sep. 2023, https://doi.org/10.3390/bioengineering10091039
-
T. Moeller, J. Krell-Roesch, A. Woll, and T. Stein, “Effects of upper-limb exoskeletons designed for use in the working environment-a literature review,” Frontiers in Robotics and AI, Vol. 9, Apr. 2022, https://doi.org/10.3389/frobt.2022.858893
-
J. L. Pons, “Rehabilitation exoskeletal robotics,” IEEE Engineering in Medicine and Biology Magazine, Vol. 29, No. 3, pp. 57–63, May 2010, https://doi.org/10.1109/memb.2010.936548
-
S. Nacy, N. Hussein, and M. Abdallh, “A review of lower limb exoskeletons,” Innovative Systems Design and Engineering, Vol. 7, No. 1, 2016.
-
N. Siddique et al., “Prototype development of an assistive lower limb exoskeleton,” in International Conference on Robotics and Automation in Industry (ICRAI), pp. 1–6, Oct. 2019, https://doi.org/10.1109/icrai47710.2019.8967351
-
G. Lv, H. Zhu, and R. D. Gregg, “On the design and control of highly back drivable lower-limb exoskeletons: a discussion of past and ongoing work,” IEEE Control Systems, Vol. 38, No. 6, pp. 88–113, Dec. 2018, https://doi.org/10.1109/mcs.2018.2866605
-
E. Shein, “Exoskeletons today,” Communications of the ACM, Vol. 62, No. 3, pp. 14–16, Feb. 2019, https://doi.org/10.1145/3303851
-
F. Molteni, G. Gasperini, G. Cannaviello, and E. Guanziroli, “Exoskeleton and end‐effector robots for upper and lower limbs rehabilitation: narrative review,” PM&R, Vol. 10, No. 9S2, Sep. 2018, https://doi.org/10.1016/j.pmrj.2018.06.005
-
D. Shi, W. Zhang, W. Zhang, and X. Ding, “A review on lower limb rehabilitation exoskeleton robots,” Chinese Journal of Mechanical Engineering, Vol. 32, No. 1, Aug. 2019, https://doi.org/10.1186/s10033-019-0389-8
-
I. Díaz, J. J. Gil, and E. Sánchez, “Lower-limb robotic rehabilitation: literature review and challenges,” Journal of Robotics, Vol. 2011, pp. 1–11, Jan. 2011, https://doi.org/10.1155/2011/759764
-
H. Y. Lee, J. H. Park, and T.-W. Kim, “Comparisons between Locomat and Walkbot robotic gait training regarding balance and lower extremity function among non-ambulatory chronic acquired brain injury survivors,” Medicine, Vol. 100, No. 18, p. e25125, May 2021, https://doi.org/10.1097/md.0000000000025125
-
K. Sirlantzis, L. B. Larsen, L. K. Kanumuru, and P. Oprea, “Robotics,” in Handbook of Electronic Assistive Technology, Elsevier, 2019, pp. 311–345, https://doi.org/10.1016/b978-0-12-812487-1.00011-9
-
“Lokomat.” Exoskeleton Report, 2024, https://exoskeletonreport.com/product/lokomat/
-
M. Dzahir and S.-I. Yamamoto, “Recent trends in lower-limb robotic rehabilitation orthosis: control scheme and strategy for pneumatic muscle actuated gait trainers,” Robotics, Vol. 3, No. 2, pp. 120–148, Apr. 2014, https://doi.org/10.3390/robotics3020120
-
“ReoAmbulator.” Exoskeleton Report, 2024, https://exoskeletonreport.com/product/reoambulator/
-
A. Esquenazi and M. Talaty, “Robotics for lower limb rehabilitation,” Physical Medicine and Rehabilitation Clinics of North America, Vol. 30, No. 2, pp. 385–397, May 2019, https://doi.org/10.1016/j.pmr.2018.12.012
-
“Walkbot.” Exoskeleton Report, 2024, https://exoskeletonreport.com/product/walkbot/
-
D. H. Kim, Y.-I. Shin, K.-L. Joa, Y. K. Shin, J. J. Lee, and S. J. H. You, “Immediate effect of Walkbot robotic gait training on neuromechanical knee stiffness in spastic hemiplegia: A case report,” NeuroRehabilitation, Vol. 32, No. 4, pp. 833–838, Jul. 2013, https://doi.org/10.3233/nre-130907
-
S.-Y. Kim et al., “Effects of innovative WALKBOT robotic-assisted locomotor training on balance and gait recovery in hemiparetic stroke: a prospective, randomized, experimenter blinded case control study with a four-week follow-up,” IEEE Transactions on Neural Systems and Rehabilitation Engineering, Vol. 23, No. 4, pp. 636–642, Jul. 2015, https://doi.org/10.1109/tnsre.2015.2404936
-
R. Olmos-Gómez, I. Calvo-Muñoz, and A. Gómez-Conesa, “Treatment with robot-assisted gait trainer Walkbot along with physiotherapy vs. isolated physiotherapy in children and adolescents with cerebral palsy. Experimental study,” BMC Neurology, Vol. 24, No. 1, Jul. 2024, https://doi.org/10.1186/s12883-024-03750-9
-
“MRG-P110.” Exoskeleton Report, 2024, https://exoskeletonreport.com/product/mrg-p110
-
A. Chien, F.-H. Hsieh, C. Huang, F.-C. Chang, N.-H. Meng, and L.-W. Chou, “The development of an emg controller-based robotic gait training system and its clinical feasibility for subacute stroke patients in improving locomotive function,” Journal of Mechanics in Medicine and Biology, Vol. 19, No. 2, p. 1940018, Apr. 2019, https://doi.org/10.1142/s0219519419400189
-
W. Liu, B. Yin, and B. Yan, “A survey on the exoskeleton rehabilitation robot for the lower limbs,” in 2nd International Conference on Control, Automation and Robotics (ICCAR), pp. 90–94, Apr. 2016, https://doi.org/10.1109/iccar.2016.7486705
-
B. Chen et al., “Recent developments and challenges of lower extremity exoskeletons,” Journal of Orthopaedic Translation, Vol. 5, pp. 26–37, Apr. 2016, https://doi.org/10.1016/j.jot.2015.09.007
-
A. Kapsalyamov, P. K. Jamwal, S. Hussain, and M. H. Ghayesh, “State of the art lower limb robotic exoskeletons for elderly assistance,” IEEE Access, Vol. 7, pp. 95075–95086, Jan. 2019, https://doi.org/10.1109/access.2019.2928010
-
N. Kh, N. Al-Hayali, J. Chiad, S. Nacy, and O. Hussein, “A review of passive and quasi-passive lower limb exoskeletons for gait rehabilitation,” Journal of Mechanical Engineering Research and Developments, Vol. 44, 2021.
-
S. Bhardwaj, A. A. Khan, and M. Muzammil, “Lower limb rehabilitation robotics: The current understanding and technology,” Work, Vol. 69, No. 3, pp. 775–793, Jul. 2021, https://doi.org/10.3233/wor-205012
-
B. Li, B. Yuan, J. Chen, Y. Zuo, and Y. Yang, “Mechanical design and human-machine coupling dynamic analysis of a lower extremity exoskeleton,” in Lecture Notes in Computer Science, Cham: Springer International Publishing, 2017, pp. 593–604, https://doi.org/10.1007/978-3-319-65289-4_56
-
D. S. Pamungkas, W. Caesarendra, H. Soebakti, R. Analia, and S. Susanto, “Overview: types of lower limb exoskeletons,” Electronics, Vol. 8, No. 11, p. 1283, Nov. 2019, https://doi.org/10.3390/electronics8111283
-
N. S. S. Sanjeevi, Y. Singh, and V. Vashista, “Recent advances in lower-extremity exoskeletons in promoting performance restoration,” Current Opinion in Biomedical Engineering, Vol. 20, p. 100338, Dec. 2021, https://doi.org/10.1016/j.cobme.2021.100338
-
B. Zhang, T. Liu, B. Zhang, and M. G. Pecht, “Recent development of unpowered exoskeletons for lower extremity: a survey,” IEEE Access, Vol. 9, pp. 138042–138056, Jan. 2021, https://doi.org/10.1109/access.2021.3115956
-
R. S. Gonçalves and L. A. O. Rodrigues, “Development of nonmotorized mechanisms for lower limb rehabilitation,” Robotica, Vol. 40, No. 1, pp. 102–119, May 2021, https://doi.org/10.1017/s0263574721000412
-
M. Srivastava, M. Srivastava, P. Sagar, and M. T. G., “Simulation of human gait for design of lower extremity exoskeletons – A review,” Materials Today: Proceedings, Vol. 44, pp. 4485–4491, Jan. 2021, https://doi.org/10.1016/j.matpr.2020.10.723
-
N. Aliman, R. Ramli, and S. M. Haris, “Design of locomotive lower limb exoskeleton with Malaysian anthropometric characteristics,” International Journal of Mechanical Engineering and Robotics Research, Vol. 8, No. 2, pp. 304–309, Jan. 2019, https://doi.org/10.18178/ijmerr.8.2.304-309
-
S. Guo, Y. Ding, and J. Guo, “Design and analysis of a lower limb exoskeleton robot,” in IEEE International Conference on Mechatronics and Automation (ICMA), pp. 1895–1900, Oct. 2020, https://doi.org/10.1109/icma49215.2020.9233806
-
M. Hu, Y. Tian, T. Liu, and M. Han, “Development of a novel lower limb rehabilitation robot in the bed,” in IEEE 18th International Conference on Industrial Informatics (INDIN), pp. 681–686, Jul. 2020, https://doi.org/10.1109/indin45582.2020.9442221
-
T. Wang et al., “A review on the rehabilitation exoskeletons for the lower limbs of the elderly and the disabled,” Electronics, Vol. 11, No. 3, p. 388, Jan. 2022, https://doi.org/10.3390/electronics11030388
-
W.-Z. Li, G.-Z. Cao, and A.-B. Zhu, “Review on control strategies for lower limb rehabilitation exoskeletons,” IEEE Access, Vol. 9, pp. 123040–123060, Jan. 2021, https://doi.org/10.1109/access.2021.3110595
-
S. Arunkumar, S. Mahesh, M. Rahul, N. Ganesh, and K. J. Maheshwaran, “Design and analysis of lower limb exoskeleton with external payload,” International Journal on Interactive Design and Manufacturing (IJIDeM), Vol. 17, No. 4, pp. 2055–2072, Mar. 2023, https://doi.org/10.1007/s12008-023-01272-1
-
A. J. Young and D. P. Ferris, “State of the art and future directions for lower limb robotic exoskeletons,” IEEE Transactions on Neural Systems and Rehabilitation Engineering, Vol. 25, No. 2, pp. 171–182, Feb. 2017, https://doi.org/10.1109/tnsre.2016.2521160
-
S. Viteckova et al., “Empowering lower limbs exoskeletons: state-of-the-art,” Robotica, Vol. 36, No. 11, pp. 1743–1756, Aug. 2018, https://doi.org/10.1017/s0263574718000693
-
M. T. Saeed, J. Z. Gul, Z. Kausar, A. M. Mughal, Z. M. U. Din, and S. Qin, “Design of model-based and model-free robust control strategies for lower limb rehabilitation exoskeletons,” Applied Sciences, Vol. 12, No. 8, p. 3973, Apr. 2022, https://doi.org/10.3390/app12083973
-
G. Bao et al., “Academic review and perspectives on robotic exoskeletons,” IEEE Transactions on Neural Systems and Rehabilitation Engineering, Vol. 27, No. 11, pp. 2294–2304, Nov. 2019, https://doi.org/10.1109/tnsre.2019.2944655
-
R. Goergen, A. C. Valdiero, L. A. Rasia, M. Oberdorfer, J. P. de Souza, and R. S. Goncalves, “Development of a pneumatic exoskeleton robot for lower limb rehabilitation,” in IEEE 16th International Conference on Rehabilitation Robotics (ICORR), pp. 187–192, Jun. 2019, https://doi.org/10.1109/icorr.2019.8779522
-
F. Hussain, R. Goecke, and M. Mohammadian, “Exoskeleton robots for lower limb assistance: A review of materials, actuation, and manufacturing methods,” Proceedings of the Institution of Mechanical Engineers, Part H: Journal of Engineering in Medicine, Vol. 235, No. 12, pp. 1375–1385, Jul. 2021, https://doi.org/10.1177/09544119211032010
-
X. Yang, H. She, H. Lu, T. Fukuda, and Y. Shen, “State of the art: bipedal robots for lower limb rehabilitation,” Applied Sciences, Vol. 7, No. 11, p. 1182, Nov. 2017, https://doi.org/10.3390/app7111182
-
G. Masengo, X. Zhang, R. Dong, A. B. Alhassan, K. Hamza, and E. Mudaheranwa, “Lower limb exoskeleton robot and its cooperative control: A review, trends, and challenges for future research,” Frontiers in Neurorobotics, Vol. 16, Jan. 2023, https://doi.org/10.3389/fnbot.2022.913748
-
Q. Fang, T. Xu, T. Zheng, H. Cai, J. Zhao, and Y. Zhu, “A rehabilitation training interactive method for lower limb exoskeleton robot,” Mathematical Problems in Engineering, Vol. 2022, pp. 1–15, Apr. 2022, https://doi.org/10.1155/2022/2429832
-
J. Zhou, S. Yang, and Q. Xue, “Lower limb rehabilitation exoskeleton robot: A review,” Advances in Mechanical Engineering, Vol. 13, No. 4, Apr. 2021, https://doi.org/10.1177/16878140211011862
-
W. M. Dos Santos, S. L. Nogueira, G. C. de Oliveira, G. G. Pena, and A. A. G. Siqueira, “Design and evaluation of a modular lower limb exoskeleton for rehabilitation,” in International Conference on Rehabilitation Robotics (ICORR), pp. 447–451, Jul. 2017, https://doi.org/10.1109/icorr.2017.8009288
-
O. Sherif, M. M. Bassuoni, and O. Mehrez, “A survey on the state of the art of force myography technique (FMG): analysis and assessment,” Medical and Biological Engineering and Computing, Vol. 62, No. 5, pp. 1313–1332, Feb. 2024, https://doi.org/10.1007/s11517-024-03019-w
-
C. Höhler, E. Trigili, D. Astarita, J. Hermsdörfer, K. Jahn, and C. Krewer, “The efficacy of hybrid neuroprostheses in the rehabilitation of upper limb impairment after stroke, a narrative and systematic review with a meta‐analysis,” Artificial Organs, Vol. 48, No. 3, pp. 232–253, Aug. 2023, https://doi.org/10.1111/aor.14618
-
S. M. Khan, A. A. Khan, and O. Farooq, “Selection of features and classifiers for EMG-EEG-based upper limb assistive devices-a review,” IEEE Reviews in Biomedical Engineering, Vol. 13, pp. 248–260, Jan. 2020, https://doi.org/10.1109/rbme.2019.2950897
-
P. K. Jamwal, S. Hussain, and M. H. Ghayesh, “Robotic orthoses for gait rehabilitation: An overview of mechanical design and control strategies,” Proceedings of the Institution of Mechanical Engineers, Part H: Journal of Engineering in Medicine, Vol. 234, No. 5, pp. 444–457, Jan. 2020, https://doi.org/10.1177/0954411919898293
-
N. Hoffmann, G. Prokop, and R. Weidner, “Methodologies for evaluating exoskeletons with industrial applications,” Ergonomics, Vol. 65, No. 2, pp. 276–295, Feb. 2022, https://doi.org/10.1080/00140139.2021.1970823
-
M. Sarajchi, M. K. Al-Hares, and K. Sirlantzis, “Wearable Lower-limb exoskeleton for children with cerebral palsy: a systematic review of mechanical design, actuation type, control strategy, and clinical evaluation,” IEEE Transactions on Neural Systems and Rehabilitation Engineering, Vol. 29, pp. 2695–2720, Jan. 2021, https://doi.org/10.1109/tnsre.2021.3136088
-
B. W. K. Ang, C.-H. Yeow, and J. H. Lim, “A critical review on factors affecting the user adoption of wearable and soft robotics,” Sensors, Vol. 23, No. 6, p. 3263, Mar. 2023, https://doi.org/10.3390/s23063263
About this article
We gratefully acknowledge the support and assistance of partners from the Rehabilitation centre in Andijan, Uzbekistan, for the work done.
The datasets generated during and/or analyzed during the current study are available from the corresponding author on reasonable request.
Each author has substantially contributed to conducting the underlying research and drafting this manuscript.
The authors declare that they have no conflict of interest.