Abstract
Congestive heart failure (CHF) is a multifaceted clinical syndrome characterized by the inability of the heart to pump blood effectively, leading to inadequate oxygen and nutrient delivery to the body tissues. Despite advancements in treatment strategies, including guideline-directed medical treatment (GDMT), end-stage CHF remains a significant cause of morbidity and mortality worldwide. Heart transplantation is considered to be the gold standard treatment of end stage CHF but constrained by the lack of organ donors, lengthening waitlists, and the negative side effects of lifelong immunosuppressive medications. Mechanical circulatory support (MCS) has emerged as a pivotal intervention for patients with end-stage CHF, serving as a bridge to recovery, transplantation, or destination therapy. The aim of this narrative review is to highlight the historical development of MCS, to assess the recent status of MCS device technology and discuss current challenges associated with complications of MCS that need to be solved in the future by device development. The history of MCS dates back to pioneering efforts in the 1960s, with significant progress in device development and utilization over decades. MCS devices, including left ventricular assist devices (LVADs), extracorporeal membrane oxygenation (ECMO), and artificial hearts, play a crucial role in providing circulatory support to patients with end-stage CHF. Recent advancements in MCS technology aim to decrease the device size, enhance blood compatibility, reduce thrombo-embolic complications, and prolong device durability and battery life and improve physiological performance of MCS. Continued research and innovation are essential to address these challenges and improve outcomes in patients with end-stage CHF. Artificial intelligence (AI) has emerged as a valuable tool in cardiovascular medicine to facilitate risk prediction, patient selection, and treatment optimization for MCS and heart transplantation. Despite these advancements, challenges persist in MCS device selection, resource allocation, and integration of AI into clinical practice. Continued research and innovation are essential to address these challenges and improve outcomes in patients with advanced heart failure.
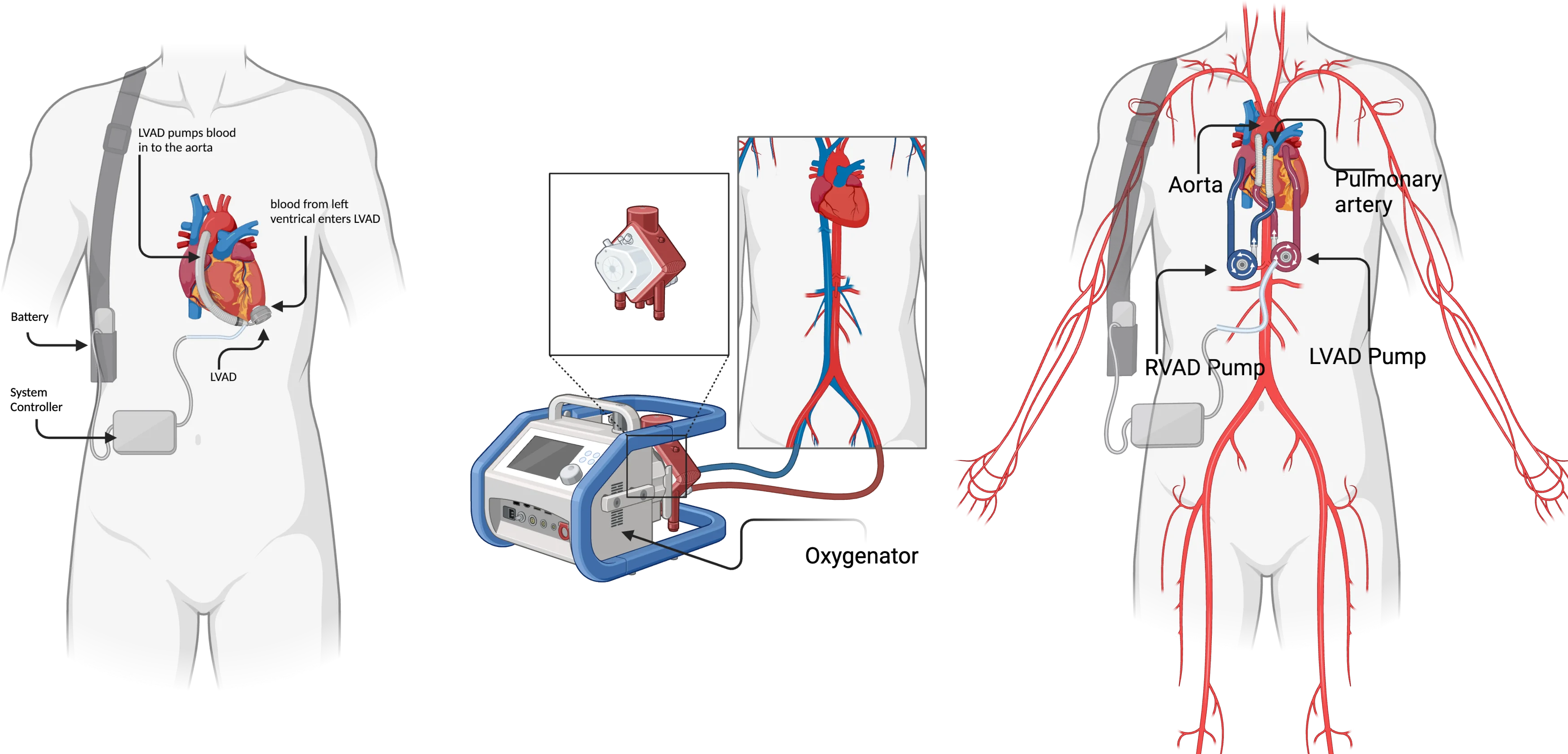
Highlights
- Biventricular assist device (BiVAD)
- Extracorporeal Membrane Oxygenation (ECMO)
- Left ventricular assist device (LVAD)
1. Introduction
Congestive heart failure (CHF) is a complex clinical syndrome resulting from the heart's inefficiency in pumping blood adequately [1]. It is a progressive disease with multiple potential causes [2]. CHF leads to the heart's inability to meet the body tissues' oxygen and nutrient requirements [2]. The condition is often associated with substantial morbidity and mortality, especially when combined with atrial fibrillation [3]. Hemodynamic congestion is a common feature in both acute decompensated heart failure with preserved and reduced ejection fraction [4]. Heart failure associated with congestion is termed congestive heart failure (CHF) [5].
Even in emerging nations, the prevalence of CHF has been steadily rising, with a forecast 46 % increase between 2012 and 2030 as a result of population aging and the longevity of patients with cardiac and noncardiac illnesses [6].
Treatment of CHF involves a multifaceted approach that includes medications and other therapies aimed at managing symptoms and improving the quality of life for individuals with this condition. End-stage CHF is a serious and potentially life-threatening condition, but with proper care and treatment, symptoms can be effectively managed [7].
During the past half century, both the causes and treatment of heart failure have changed considerably [8]. Survival rates for patients with systolic heart failure have improved over the past 20 years, although the prognosis for diastolic heart failure has not seen significant changes [9]. The prognosis for heart failure remains dismal, with a high mortality rate during the first year of diagnosis and a significant economic effect, despite improvements in medical therapy and a minor improvement in overall survival rates [10].
Despite marked improvements in CHF management over the past three decades, about 50 % of patients still die within five years of diagnosis, indicating that important pathogenic mechanisms are not fully addressed by current treatments [11]. Cardiac transplantation is currently the only effective treatment for end-stage CHF. However, numbers are constrained by the lack of organ donors, lengthening waitlists that increase mortality, and the negative side effects of lifelong immunosuppressive medications.
Mechanical circulatory support (MCS) is a treatment used to support heart function in patients with end-stage CHF. By supporting blood circulation, MCS devices restore heart function and help the patient maintain vital functions [12]. These devices are designed to be used on a permanent basis, often while waiting for a heart transplant as a bridge to transplantation and as a final form of therapy. MCS can improve organ perfusion and function in patients with heart failure, improving quality of life and patient prognosis [12]. Therefore, MCS can also be used as a targeted therapy in patients who are not candidates for heart transplantation [13].
This narrative review aims to explore the historical course of MCS devices and to assess the current opportunities and challenges for a better application.
2. The history and status of mechanical circulatory support (MCS)
The National Institute of Health initiated its artificial heart program in 1964 to advance the development of both partial and complete cardiac replacement systems [14]. This initiative coincided with efforts in Cleveland and Texas to pioneer circulatory replacement systems. Notably, at Baylor University in Houston, Texas, William DeBakey performed the first human implantation of a left ventricular assist device (LVAD) in 1963 (Fig. 1), although the patient succumbed four days later [14]. In 1969, Denton Cooley sustained a human patient on support for 64 hours before transplantation, but unfortunately, the patient passed away 32 hours post-transplant due to pneumonia [14]. Cooley’s pump, featuring two chambers and an external pneumatic driver, operated on the same principle as contemporary designs. Twelve years later, Cooley refined his pump and implanted a second artificial heart, a pneumatic dual-chambered Akutsu Total Artificial Heart (TAH), with the patient surviving 39 hours post-implant [14].
Fig. 1Left ventricular assist device (LVAD), adapted from UCI Health [15], by BioRender.com (2023)
![Left ventricular assist device (LVAD), adapted from UCI Health [15], by BioRender.com (2023)](https://static-01.extrica.com/articles/24174/24174-img1.jpg)
Public attention was first drawn to MCS with the successful implantation of the Jarvik-7 total artificial heart (TAH) (Fig. 2) in a patient in December 1982. The device marked a milestone in cardiac assistive technology, sparking further interest and development in the field. This was followed by the increasing usage of left ventricular assist devices (LVADs) in the 1990s to provide temporary support for patients as they awaited heart transplants [16].
Fig. 2Jarvik-7 total artificial heart [17]
![Jarvik-7 total artificial heart [17]](https://static-01.extrica.com/articles/24174/24174-img2.jpg)
Recent studies have highlighted the importance of early MCS implantation in patients with cardiogenic shock, as initial vasopressor and inotropic therapies may not be sufficient to stabilize the patient’s condition [18]. The use of temporary circulatory support systems, including MCS devices, has increased significantly in recent years, despite being resource-intensive and associated with major complications [19].
In cases of acute circulatory collapse or fulminant myocarditis, MCS has been utilized to keep patients alive until intrinsic cardiac function recovers sufficiently to remove the support device [20]. For patients with acute dilated cardiomyopathy in the setting of suspected myocarditis, standard heart failure treatment combined with MCS has shown improvements in ventricular function and clinical status [21].
The development of MCS devices has been driven by the need to support patients with end-stage CHF as a bridge to cardiac transplantation, recovery, or as an alternative to transplantation [22]. The Interagency Registry for Mechanical Circulatory Support (INTERMACS) has played a crucial role in advancing the field by collecting data and enabling risk-adjusted outcomes measurement [23]. Over the years, there has been a rapid pace of development in MCS devices, with the goal of improving patient outcomes and providing effective circulatory support [24].
Various MCS devices, such as intra-aortic balloon pumps, Impella®, and extracorporeal membrane oxygenation, have been utilized to provide circulatory support and act as a bridge to recovery, durable support, or cardiac transplantation [25]. These devices have been instrumental in managing conditions like cardiogenic shock due to myocarditis, where MCS may be required to bridge the patient to recovery or heart transplantation [26].
The evolution of ventricular assist devices (VADs) from first-generation pulsatile pumps to second- and third-generation continuous-flow devices has been driven by the need to overcome the limitations of early devices. First-generation VADs, with their pulsatile flow design, were characterized by being large, cumbersome, and noisy, leading to suboptimal outcomes primarily due to durability issues and technical challenges [27]. This prompted the development of second- and third-generation VADs, such as continuous-flow devices, which offered improvements in size, biocompatibility, durability, and effectiveness [28]. The transition to continuous-flow devices was further supported by the recognition of their enhanced durability, smaller sizes, and better survival rates compared to pulsatile-flow devices [29].
The pivotal Randomized Evaluation of Mechanical Assistance for the Treatment of Congestive Heart Failure (REMATCH) study, conducted in 2001, played a crucial role in establishing durable Mechanical Circulatory Support (MCS) as a permanent treatment option for patients with end-stage heart failure who were not eligible for heart transplantation. This study transformed the perception of MCS by demonstrating its potential as a long-term solution rather than just a temporary measure [30]. The REMATCH trial showed that first-generation ventricular assist devices (VADs) significantly improved patient survival rates and quality of life compared to those solely on medical therapy. However, it also underscored that these devices were associated with a notable number of adverse events [30].
Advancements in ventricular assist device technology have led to the transition from pulsatile-flow VADs to continuous-flow left ventricular assist devices (CF-LVADs), which have enhanced device design and functionality [28]. These advancements have contributed to improving patient outcomes and quality of life in individuals with advanced heart failure [31]. Despite progress in device design, concerns such as thrombosis remain, emphasizing the necessity for ongoing research and innovation in the field of mechanical circulatory support [32].
The utilization of MCS devices, including VADs, has become increasingly prevalent in managing acute and chronic ventricular failure, highlighting the importance of these devices in modern cardiac care [33]. While VADs offer significant benefits in terms of patient survival and quality of life, they also pose challenges such as infections and thromboembolic events, necessitating continuous monitoring and management [34]. Additionally, the introduction of novel technologies like minimally invasive approaches for VAD implantation and innovative cannulation techniques reflects the ongoing efforts to enhance outcomes and reduce complications associated with these devices [35], [36].
3. Mechanical circulatory support (MCS) technology
In clinical practice, percutaneous mechanical circulatory support devices like Impella®, Extracorporeal Membrane Oxygenation (ECMO) (Fig. 3), and TandemHeart™ have become valuable tools for providing hemodynamic support to patients [37]. These devices have shown effectiveness in improving outcomes for patients with cardiogenic shock and advanced heart failure [38]. Additionally, the use of continuous-flow left ventricular assist devices has demonstrated significant benefits in improving hemodynamic support and quality of life for patients awaiting heart transplantation [39].
The selection of mechanical circulatory support devices is crucial, especially in cases of severe biventricular failure. Options such as total artificial hearts, contemporary left ventricular assist devices (LVADs) coupled with right ventricular assist devices (RVADs), and biventricular assist devices have shown promise in managing such complex cases [40]. Furthermore, the use of mechanical circulatory support devices in acute myocardial infarction and cardiogenic shock has been supported by guidelines, highlighting their importance as adjunctive therapies [41].
Fig. 3Extracorporeal Membrane Oxygenation (ECMO), adapted from Soltes at al. [42] by BioRender.com (2023)
![Extracorporeal Membrane Oxygenation (ECMO), adapted from Soltes at al. [42] by BioRender.com (2023)](https://static-01.extrica.com/articles/24174/24174-img3.jpg)
Classification of mechanical circulatory support devices (Fig. 4) encompasses a range of technologies designed to assist or replace heart function in patients with various cardiac conditions. These devices play a crucial role in supporting circulation and maintaining adequate perfusion.
Fig. 4Classification of mechanical circulatory support devices [43]
![Classification of mechanical circulatory support devices [43]](https://static-01.extrica.com/articles/24174/24174-img4.jpg)
Some of the key mechanical circulatory support devices include:
1) BiVAD (Biventricular Assist Device): Provides support to both the left and right ventricles (Fig. 5).
2) ECMO (Extracorporeal Membrane Oxygenation): Offers temporary support by oxygenating the blood outside the body.
3) LVAD (Left Ventricular Assist Device): Supports the left ventricle's function.
4) PVAD (Pulsatile Ventricular Assist Device): Aids in ventricular function through pulsatile support.
5) RVAD (Right Ventricular Assist Device): Specifically supports the right ventricle.
6) VAD (Ventricular Assist Device): Generally, refers to devices that assist one or both ventricles.
Various specific devices fall under these categories, such as the BVS 5000 (ABIOMED Inc, USA), CentriMag™, EvaHeart®, HeartAssist5®, HeartMate II™, HeartWare™ HVAD, Impella®, Incor(Berlin Heart AG, Germany), Jarvik® 2000, Rotaflow (Maquet Holding BV & Co.), TandemHeart™, and VentriAssist (Ventracor, Ltd). These devices serve different purposes, from short-term circulatory support to long-term solutions like destination therapy or bridge-to-transplant [43].
The classification of Mechanical Circulatory Support (MCS) is crucial for understanding the disease state and guiding treatment strategies to enhance patient outcomes. One notable classification system is proposed by the Interagency Registry for Mechanically Assisted Circulatory Support (INTERMACS), which defines seven clinical profiles to evaluate disease status, risk of implantation of mechanical circulatory support devices (MCSDs), and the appropriate timing for intervention [44]. This classification system offers a convenient framework for characterizing the severity of heart failure in patients undergoing treatment with MCS.
Third-generation LVADs, exemplified by devices like the HeartWare™ ventricular assist device (HVAD) and the HeartMate III, have introduced significant enhancements to mechanical circulatory support technology. The HVAD, with its centrifugal pump design, offered the potential for less invasive intrapericardial implantation and demonstrated improved patient outcomes, including enhanced survival rates at one year and improved functional capacity and quality of life. Despite risks such as stroke, right heart failure, and sepsis, the HVAD was considered non-inferior to the HeartMate II™ and received FDA approval in 2012 [45].
Fig. 5Biventricular Assist Device, Adapted from “Biventricular assist device (BiVAD)” by BioRender.com (2023) [46]
![Biventricular Assist Device, Adapted from “Biventricular assist device (BiVAD)” by BioRender.com (2023) [46]](https://static-01.extrica.com/articles/24174/24174-img5.jpg)
The HeartMate™ III, the latest advancement in third-generation LVADs, features a centrifugal continuous flow design with a fully magnetically levitated impeller, eliminating contact points and reducing wear and tear. This device showcased impressive outcomes in the MOMENTUM 3 study, notably eliminating the risk of pump thrombosis. The design of the HeartMate™ III, incorporating a relatively large impeller housing and automated rotational speed variation to create some degree of “pulsatility”, has shown superior results in terms of survival free from disabling stroke or the need for device replacement at two years post-implantation. The HeartMate™ III received CE Mark approval in 2015 [47].
Innovations in surgical techniques have proposed less invasive methods for HeartMate™ III implantation, preserving pericardial integrity and potentially reducing short-term procedural complications and long-term right heart failure. While these advancements represent significant progress in the field of mechanical circulatory support, further research is necessary to validate the potential benefits of these innovations [48].
The evolution of LVAD technology from first-generation devices to the current third-generation devices like the HeartWare™ HVAD and HeartMate III underscores the continuous improvement in device design and patient outcomes. These advancements have the potential to enhance the quality of life and survival rates of individuals with advanced heart failure, highlighting the importance of ongoing research and innovation in the field of mechanical circulatory support.
4. Total artificial heart technology
Total Artificial Heart (TAH) technology represents a significant advancement in the field of mechanical circulatory support, offering a biventricular assist device that can serve as a bridge to transplant or as a destination therapy. The TAH has been studied for its effectiveness in managing heart failure patients and has shown promise in improving patient outcomes [49]. Third-generation TAHs, such as the HeartWare™ ventricular assist device (HVAD) and the HeartMate™ III, have introduced innovative features to enhance patient care. The HVAD, with its centrifugal pump design, allowed for less invasive intrapericardial implantation and demonstrated improved patient survival rates and quality of life. Despite associated risks like stroke and sepsis, the HVAD was considered non-inferior to other devices and received FDA approval [50]. The HeartMate™ III, another third-generation TAH, features a centrifugal continuous flow design with a fully magnetically levitated impeller, reducing wear and tear and eliminating the risk of pump thrombosis. This device has shown superior outcomes in terms of survival and freedom from disabling stroke or device replacement, highlighting its potential as an advanced mechanical circulatory support option [51].
The SynCardia Systems, LLC, Total Artificial Heart (TAH) is a groundbreaking mechanical circulatory support device that has received FDA [52]. Developed in Tucson, Arizona, this innovative technology consists of four chambers, four tilting disc valves, and cannulas connected to an external pneumatic driver to mimic the pulsatile flow of a natural heart. The SynCardia Systems, LLC, TAH has played a pivotal role in offering biventricular support and has been sanctioned as a bridge-to-transplant strategy in multiple regions, including Canada, the United States, and Europe [53]. This device has proven to be effective in managing heart failure patients, providing a viable option for individuals awaiting heart transplantation.
One of the distinctive features of the SynCardia Systems, LLC, TAH is its flexible polyurethane ventricles. This flexibility provides surgeons greater freedom during implantation, enabling a more suitable fit for individual patients. The design involves an expandable polyurethane diaphragm that separates the chambers carrying blood from those filled with air. The external pneumatic drivers are the engine of this device. They generate pneumatic pulses that inflate the expandable diaphragms and displace the volume inside the ventricles. This process causes blood to be ejected into the pulmonary and systemic circulatory systems, emulating the function of a biological heart.
The SynCardia Systems, LLC, Total Artificial Heart (TAH) has had a significant impact in the field of mechanical circulatory support, with over 1300 implants globally. In North America, 373 artificial heart implants have been documented in the INTERMACS database, a registry of data for adults who have received mechanical circulatory support devices due to heart failure. For patients under 19 years old dependent on mechanical circulatory support, the PEDIMACS registry, the pediatric portion of INTERMACS, reports that 2 % are TAH recipients [52]. The SynCardia TAH has been a pivotal device in providing biventricular support and has been utilized as a bridge-to-transplant strategy in various regions worldwide. This device has demonstrated effectiveness in managing heart failure patients, offering a viable option for individuals awaiting heart transplantation [53].
The continuous evolution and utilization of the SynCardia Systems, LLC, TAH underscore its importance in improving patient care and outcomes, particularly for those with severe biventricular heart failure. The device’s ability to provide comprehensive circulatory support by replacing both ventricles and cardiac valves has significantly contributed to enhancing patient outcomes and serving as a bridge to heart transplantation for individuals with end-stage heart failure [54].
The Carmat Total Artificial Heart (TAH), with a unique design called Aeson® that combines prosthetic components with bovine parts, was developed by CARMAT in France. This innovative device comprises two chambers that separate hydraulic fluid from blood using flexible bio membranes. The hydraulic fluid generates force to propel the blood, while the bovine pericardium used in the membrane that interfaces with the blood enhances biocompatibility. However, concerns about mechanical durability have been raised [55]. The Carmat TAH system incorporates a self-modulating feature that adjusts cardiac output based on the patient's activity level, providing a personalized response to varying physiological demands. Clinical trials of the device have shown promising results, with the first patient surviving for 75 days in December 2013 [56]. The development of the Carmat TAH was spearheaded by Dr. Alain Carpentier, a renowned French surgeon recognized as a pioneer in modern mitral valve repair [57].
While the Carmat TAH demonstrates reduced platelet adhesion and blood cell deposition on the membrane, the use of bovine pericardium raises concerns regarding mechanical durability. The system’s ability to modulate cardiac output based on the patient's activity level represents a significant advancement in TAH technology, offering a more adaptive and responsive approach to circulatory support.
The BiVACOR® Total Artificial Heart (TAH) developed by BiVACOR® in Houston, Texas, represents a significant advancement in mechanical circulatory support technology. This innovative device is a centrifugal flow pump capable of delivering up to 12 L/min of cardiac output while featuring just two moving parts: an internal axial rotor that is entirely magnetically suspended to withstand wear and tear. The BiVACOR® TAH is designed to automatically respond to and adapt to the continuously changing flow requirements of the human body. Both in-vitro and in-vivo investigations have demonstrated that the device can operate with pulsatile flow dynamics, showcasing its adaptability and effectiveness [58]. One of the key distinguishing features of the BiVACOR® TAH is its compact size, enabling its use in both adults and children without the need for separate versions. This versatility makes the BiVACOR® TAH a promising option for a wide range of patients with end-stage heart failure. The device's ability to modulate cardiac output based on the patient's activity level represents a significant advancement in TAH technology, offering a more personalized and responsive approach to circulatory support [59].
Currently in the pre-clinical phase of development, the BiVACOR® TAH holds great promise in the field of mechanical circulatory support. Ongoing research and refinement of the device aim to optimize its performance, durability, and biocompatibility, with the ultimate goal of providing a durable and effective alternative to heart transplantation for individuals with severe heart failure.
HybridHeart, a groundbreaking technology in the field of artificial hearts, aims to replicate the contraction of the heart's muscles using artificial muscle technology. Bas Overvelde, an expert in soft robotics at Eindhoven University of Technology, describes the artificial heart as a system of complex balloons. The device developed by the company features an internal chamber that holds blood, and when the balloons are inflated, the internal chamber contracts and pumps the blood, mimicking the natural pulsating flow of the heart [60]. The development of artificial muscle technology is crucial for the advancement of soft robotics, as it aims to replicate the versatility, performance, and reliability of biological muscles. Artificial muscles play a vital role in soft robots, mimicking the muscles found in biological systems and enabling a wide range of applications in robotics [61].
Artificial muscles powered soft robotic devices have the potential to mimic complex biological systems such as heart compression and twisting. These advancements in artificial muscle technology enable the creation of innovative devices like HybridHeart, which can replicate the intricate motions of biological tissues [62]. The integration of artificial muscle technology in soft robotics allows for the development of devices that can achieve complex motions, similar to those of human tissues. By utilizing artificial muscles, soft robots can perform functions that mimic the movements of biological systems, offering new possibilities in the field of robotics [63].
In conclusion, the development of artificial muscle technology is a key driver in the advancement of soft robotics and artificial heart technology. By replicating the capabilities of biological muscles, artificial muscles enable the creation of innovative devices like HybridHeart, which have the potential to revolutionize the field of mechanical circulatory support.
5. Mechanical circulatory support (MCS) device selection
The selection of MCS devices is based on factors such as pump size, biocompatibility, durability, effectiveness, and susceptibility to infection [64]. Guidelines from organizations like the American College of Cardiology and the American Heart Association are crucial in guiding the selection process for MCS devices in heart failure patients [65], [66].
The European Society of Cardiology offers valuable insights into advanced heart failure, stressing the significance of accurately defining advanced heart failure to determine suitable treatments like heart transplantation or long-term MCS devices [67], [68]. Long-term MCS is underscored as a pivotal treatment modality for individuals with severe heart failure [38]. The evolution of permanent implantable MCS systems has addressed critical aspects such as pump size, biocompatibility, durability, and susceptibility to infection [64], [68].
The revised heart organ allocation policy by the United Network for Organ Sharing (UNOS) has led to significant changes in the landscape of heart transplantation and mechanical circulatory support (MCS) devices. Following the implementation of the 2018 heart allocation policy change, there has been a noticeable increase in the use of temporary MCS devices as a bridge to transplantation for patients with cardiogenic shock [69]. This shift has made bridging with durable MCS devices more challenging, necessitating routine assessments of patients supported on temporary MCS devices for potential myocardial recovery before urgent transplantation [70].
The evolving trends in adult heart transplant following the 2018 heart allocation policy change have shown improvements in posttransplant survival rates, indicating a learning curve that transplant centers have experienced with refined patient selection under the new policy [71]. Furthermore, modifications to the current heart allocation policy may be necessary to facilitate the bridging of patients with durable left ventricular assist devices [72].
Artificial intelligence (AI) has become a valuable tool in cardiovascular medicine, particularly in predicting outcomes for patients undergoing durable mechanical circulatory support (MCS) and heart transplantation (HT) for end-stage heart failure [73]. The integration and analysis of complex clinical data using AI have shown promise in improving risk prediction and optimizing patient selection for these therapies [74], [75], [76], [77], [78]. By leveraging AI algorithms that learn iteratively from data, computers can uncover hidden insights without explicit programming, enabling the identification of key variables such as comorbidities, laboratory values, echocardiogram findings, and biomarkers that influence treatment outcomes [75], [76], [77].
Recent studies have highlighted the potential of AI in revolutionizing risk prediction and stratification, diagnostics, precision medicine, workflows, and efficiency in cardiology, emphasizing its role in enhancing resource allocation and reducing complications like mortality [74]. AI models can not only prognosticate perioperative risks but also assist in selecting the most suitable candidates for durable MCS or HT based on preoperative variables, thereby maximizing the benefits of these therapies [76], [77].
Moreover, the application of AI in cardiovascular medicine extends beyond risk prediction to encompass areas such as the development of AI-enabled models for early detection of conditions like left ventricular hypertrophy (LVH) and mortality prediction in young to middle-aged adults using electrocardiograms (ECGs) [79]. AI has also been instrumental in predicting patient age from ECGs, offering a potential biomarker for cardiovascular age that correlates with mortality and comorbidities [80].
While AI holds great promise in advancing cardiovascular medicine, it is essential to critically evaluate AI-based prediction models to ensure their reliability and effectiveness in clinical practice [78], [81]. The field of AI in medicine is rapidly evolving, with ongoing research focusing on enhancing the accuracy and interpretability of AI models for improved decision-making in disease management.
6. Current limitations of MCS including TAH
The management of patients with mechanical circulatory support (MCS) devices presents challenges, with one primary limitation being the increased risk of thromboembolic events. The presence of mechanical parts and artificial surfaces within MCS devices can lead to blood clot formation, potentially resulting in severe complications such as stroke or systemic embolism. While advancements in anticoagulant techniques have reduced these risks, preventing thrombosis remains a complex task. Balancing the need for anticoagulation to prevent clot formation and the risk of bleeding is crucial. Excessive bleeding can lead to hemodynamic instability and adverse outcomes, while inadequate anticoagulation can result in thromboembolic events [82], [83].
Addressing the challenges associated with anticoagulation in MCS patients requires a comprehensive approach that considers individual patient factors, device-specific considerations, and the delicate balance between preventing thrombosis and minimizing bleeding risks. Centralized anticoagulation management involving a collaborative effort between healthcare providers and specialized services can help optimize anticoagulation strategies and improve patient outcomes [84].
Moreover, the development of personalized anticoagulation protocols tailored to the specific needs of MCS patients is essential to minimize the risks of thrombosis and bleeding complications. Complementary tests that assess coagulation status, platelet function, and heparin-effect can help individualize anticoagulation management and optimize outcomes for patients receiving mechanical circulatory support [85].
Another limitation is the size and compatibility of current mechanical circulatory support (MCS) devices pose significant challenges, limiting the number of eligible candidates and complicating the implantation process. The large diameters of today's Total Artificial Hearts (TAHs) may not be suitable for all patients due to anatomical constraints. The substantial size of these devices necessitates extensive mediastinal exploration and reoperations, leading to increased risks of bleeding and complications during implantation [86]. The size limitation of MCS devices, particularly TAHs, presents obstacles in achieving optimal patient outcomes. The large size of these devices may restrict their implantation in patients with smaller anatomies, posing challenges during surgical procedures. Additionally, the substantial dissection required for device installation can lead to increased risks of bleeding, mediastinal exploration, and the need for reoperations, further complicating the management of patients with severe heart failure [87].
The requirement for a constant power supply is a significant drawback for patients with mechanical circulatory support (MCS) devices, as it often involves external batteries or power sources. This necessity can pose logistical challenges for patients, limiting their mobility and potentially impacting their quality of life. However, advancements in technology offer hope for improved treatment processes. For instance, researchers from the Real Heart company are developing a new generation of artificial hearts that address the issue of power supply. The need for a constant power supply presents a significant challenge for patients with mechanical circulatory support (MCS) devices, often relying on external batteries or power sources. This requirement can create logistical hurdles for patients, limiting their mobility and potentially affecting their quality of life. Nevertheless, advancements in technology provide promise for improved treatment processes. For example, researchers at Real Heart company are working on a new generation of artificial hearts to tackle the power supply issue [88].
The development of artificial hearts addressing power supply concerns signifies a notable advancement in the field of mechanical circulatory support. By concentrating on innovative solutions to ensure continuous power availability for MCS devices, researchers strive to enhance patient mobility, quality of life, and overall treatment outcomes. These advancements have the potential to transform the management of patients with severe heart failure and enhance their overall well-being.
The final limitations of these devices are their lifespan and durability. The constant use of these devices raises questions about their long-term endurance, especially considering the mechanical strains they endure when in use.
7. Conclusions
The landscape of heart failure management has undergone a profound transformation, largely driven by the increasing utilization of MCS devices. These devices serve as vital lifelines for patients facing end-stage CHF and cardiogenic shock, offering a spectrum of interventions ranging from bridge-to-transplant to destination therapy and temporary support. Studies have highlighted the lifesaving potential of MCS devices, demonstrating their effectiveness in improving outcomes for individuals with end-stage heart failure.
The evolution of permanent implantable MCS systems has significantly enhanced the prognosis for individuals with severe heart failure, offering a pathway to transplantation or serving as long-term destination therapy.
The quest for viable and functional mechanical replacements for failing hearts has been a formidable challenge in modern medicine, driven by the scarcity of biological organs. The development of MCS represents a culmination of efforts to address this challenge. Blood compatibility emerges as a critical area in biomaterial science, although the quest for fully hemocompatible materials remains ongoing. Moreover, innovations in energy sources, such as transcutaneous energy, aim to overcome limitations associated with driveline-derived diseases.
While heart transplantation remains the gold standard of care in the foreseeable future, researchers are actively exploring novel technologies to enhance graft preservation and expand the donor pool. Tissue engineering and cardiovascular regenerative medicine are at the forefront of efforts to develop alternative treatments for heart failure. Cell-based techniques and biomaterials capable of supporting cardiac regeneration show promise, with the ultimate goal of bioengineering a fully biocompatible, individualized replacement for the failing heart using decellularized native cardiac extracellular matrix and patient-derived cardiovascular cells.
References
-
A. C. Bales and M. J. Sorrentino, “Causes of congestive heart failure,” Postgraduate Medicine, Vol. 101, No. 1, pp. 44–56, Jun. 2015, https://doi.org/10.3810/pgm.1997.01.141
-
A. Pourtaji, V. Jahani, S. M. H. Moallem, A. Karimani, and A. H. Mohammadpour, “Application of G-CSF in congestive heart failure treatment,” Current Cardiology Reviews, Vol. 15, No. 2, pp. 83–90, Mar. 2019, https://doi.org/10.2174/1573403x14666181031115118
-
D. Roy et al., “Rhythm control versus rate control for atrial fibrillation and heart failure,” New England Journal of Medicine, Vol. 358, No. 25, pp. 2667–2677, Jun. 2008, https://doi.org/10.1056/nejmoa0708789
-
L. N. L. van Aelst et al., “Acutely decompensated heart failure with preserved and reduced ejection fraction present with comparable haemodynamic congestion,” European Journal of Heart Failure, Vol. 20, No. 4, pp. 738–747, Dec. 2017, https://doi.org/10.1002/ejhf.1050
-
J. Cops, S. Haesen, B. de Moor, W. Mullens, and D. Hansen, “Current animal models for the study of congestion in heart failure: an overview,” Heart Failure Reviews, Vol. 24, No. 3, pp. 387–397, Jan. 2019, https://doi.org/10.1007/s10741-018-9762-4
-
A. Groenewegen, F. H. Rutten, A. Mosterd, and A. W. Hoes, “Epidemiology of heart failure,” European Journal of Heart Failure, Vol. 22, No. 8, pp. 1342–1356, Jun. 2020, https://doi.org/10.1002/ejhf.1858
-
A. Mosterd and A. W. Hoes, “Clinical epidemiology of heart failure,” Heart, Vol. 93, No. 9, pp. 1137–1146, Sep. 2007, https://doi.org/10.1136/hrt.2003.025270
-
E. Braunwald and M. R. Bristow, “Congestive heart failure: fifty years of progress,” Circulation, Vol. 102, Nov. 2000, https://doi.org/10.1161/circ.102.suppl_4.iv-14
-
W. H. Gaasch and W. C. Little, “Assessment of left ventricular diastolic function and recognition of diastolic heart failure,” Circulation, Vol. 116, No. 6, pp. 591–593, Aug. 2007, https://doi.org/10.1161/circulationaha.107.716647
-
J. J. Mcmurray, “Heart failure: epidemiology, aetiology, and prognosis of heart failure,” Heart, Vol. 83, No. 5, pp. 596–602, May 2000, https://doi.org/10.1136/heart.83.5.596
-
W. Li et al., “Gut microbiota‐derived trimethylamine N ‐oxide is associated with poor prognosis in patients with heart failure,” Medical Journal of Australia, Vol. 213, No. 8, pp. 374–379, Sep. 2020, https://doi.org/10.5694/mja2.50781
-
B. Szyguła-Jurkiewicz, W. Szczurek, K. Suliga, G. Rempega, and P. Rajwa, “Mechanical circulatory support in heart failure,” Polish Journal of Cardio-Thoracic Surgery, Vol. 2, No. 2, pp. 130–134, Jan. 2016, https://doi.org/10.5114/kitp.2016.61046
-
L. A. Hajjar and J.-L. Teboul, “Mechanical circulatory support devices for cardiogenic shock: state of the art,” Critical Care, Vol. 23, No. 1, Mar. 2019, https://doi.org/10.1186/s13054-019-2368-y
-
H. Martinez, N. R. Alberson, J. J. Guerra, and I. A. Salas de Armas, “A historical review of mechanical circulatory support,” in Ventricular Assist Devices – Advances and Applications in Heart Failure, IntechOpen, 2023, https://doi.org/10.5772/intechopen.110525
-
“UCI health first in orange county to offer ventricular assist device for patients with end-stage heart failure.” UCI Health, 2020, https://www.ucihealth.org/news/2020/04/first-in-orange-county-to-offer-left-ventricular-assist-device/
-
S. Khan and W. Jehangir, “Evolution of artificial hearts: an overview and history,” Cardiology Research, Vol. 5, No. 5, pp. 121–125, Jan. 2014, https://doi.org/10.14740/cr354w
-
“Jarvik 7 – an artificial heart.” Science Museum Blog, 2017, https://blog.sciencemuseum.org.uk/jarvik-7-an-artificial-heart/
-
R. Muñoz-Rodríguez et al., “Ultrasound assessment in cardiogenic shock weaning: a review of the state of the art,” Journal of Clinical Medicine, Vol. 10, No. 21, p. 5108, Oct. 2021, https://doi.org/10.3390/jcm10215108
-
A. Combes, S. Price, A. S. Slutsky, and D. Brodie, “Temporary circulatory support for cardiogenic shock,” The Lancet, Vol. 396, No. 10245, pp. 199–212, Jul. 2020, https://doi.org/10.1016/s0140-6736(20)31047-3
-
A. Kavoliunienė, E. Ruzgytė, and L. Jankauskienė, “Use of mechanical circulatory support in acute circulatory collapse at immediate risk of death after endomyocardial biopsy: a case report,” Cardiometry, No. 3, pp. 118–123, Nov. 2013.
-
S. Sagar, P. P. Liu, and L. T. Cooper, “Myocarditis,” The Lancet, Vol. 379, No. 9817, pp. 738–747, Feb. 2012, https://doi.org/10.1016/s0140-6736(11)60648-x
-
M. C. Deng, “Mechanical circulatory support device database of the international society for heart and lung transplantation,” Current Opinion in Cardiology, Vol. 18, No. 2, pp. 147–152, Mar. 2003, https://doi.org/10.1097/00001573-200303000-00014
-
W. L. Holman, S. V. Pamboukian, D. C. Mcgiffin, J. A. Tallaj, M. Cadeiras, and J. K. Kirklin, “Device related infections: are we making progress?,” Journal of Cardiac Surgery, Vol. 25, No. 4, pp. 478–483, Apr. 2010, https://doi.org/10.1111/j.1540-8191.2010.01034.x
-
L. Hubbert, P. Sundbom, M. Loebe, B. Peterzén, H. Granfeldt, and H. Ahn, “Acoustic analysis of a mechanical circulatory support,” Artificial Organs, Vol. 38, No. 7, pp. 593–598, Dec. 2013, https://doi.org/10.1111/aor.12244
-
S. M. Fernando, S. Price, R. Mathew, A. S. Slutsky, A. Combes, and D. Brodie, “Mechanical circulatory support in the treatment of cardiogenic shock,” Current Opinion in Critical Care, Vol. 28, No. 4, pp. 434–441, Jul. 2022, https://doi.org/10.1097/mcc.0000000000000956
-
I. Kindermann et al., “Update on myocarditis,” Journal of the American College of Cardiology, Vol. 59, No. 9, pp. 779–792, Feb. 2012, https://doi.org/10.1016/j.jacc.2011.09.074
-
S. Crow et al., “Gastrointestinal bleeding rates in recipients of nonpulsatile and pulsatile left ventricular assist devices,” The Journal of Thoracic and Cardiovascular Surgery, Vol. 137, No. 1, pp. 208–215, Jan. 2009, https://doi.org/10.1016/j.jtcvs.2008.07.032
-
L. K. Truby et al., “Ventricular assist device utilization in heart transplant candidates,” Circulation: Heart Failure, Vol. 11, No. 4, Apr. 2018, https://doi.org/10.1161/circheartfailure.117.004586
-
G. P. Itkin, A. S. Bychnev, A. P. Kuleshov, and A. A. Drobyshev, “Haemodynamic evaluation of the new pulsatile-flow generation method in vitro,” The International Journal of Artificial Organs, Vol. 43, No. 3, pp. 157–164, Oct. 2019, https://doi.org/10.1177/0391398819879939
-
J. C. Fang, “Rise of the machines – left ventricular assist devices as permanent therapy for advanced heart failure,” New England Journal of Medicine, Vol. 361, No. 23, pp. 2282–2285, Dec. 2009, https://doi.org/10.1056/nejme0910394
-
K. L. Wood et al., “Complete sternal-sparing heartmate 3 implantation: a case series of 10 consecutive patients,” The Annals of Thoracic Surgery, Vol. 107, No. 4, pp. 1160–1165, Apr. 2019, https://doi.org/10.1016/j.athoracsur.2018.10.005
-
K. A. Kortekaas et al., “Left ventricular assist device and pump thrombosis: the importance of the inflow cannula position,” The International Journal of Cardiovascular Imaging, Vol. 38, No. 12, pp. 2771–2779, Jul. 2022, https://doi.org/10.1007/s10554-022-02683-z
-
M. E. Stone, “Current status of mechanical circulatory assistance,” Seminars in Cardiothoracic and Vascular Anesthesia, Vol. 11, No. 3, pp. 185–204, Aug. 2016, https://doi.org/10.1177/1089253207306093
-
R. Z. Olmsted et al., “Severe lvad-related infections requiring surgical treatment: incidence, predictors, effect on survival, and impact of device selection,” Journal of Cardiac Surgery, Vol. 34, No. 2, pp. 82–91, Feb. 2019, https://doi.org/10.1111/jocs.13987
-
W. Z. Chancellor, C. Tribble, R. Ghanta, and L. T. Yarboro, “Novel cannulation technique for temporary right ventricular assist device after lvad placement,” Operative Techniques in Thoracic and Cardiovascular Surgery, Vol. 23, No. 2, pp. 90–100, Jan. 2018, https://doi.org/10.1053/j.optechstcvs.2018.12.001
-
M. C. Mongé, E. Kalb, S. Ramlogan, and A. Joong, “Interventricular septal hematoma complicating ventricular assist device placement in an infant: case report and review of the literature,” World Journal for Pediatric and Congenital Heart Surgery, Vol. 14, No. 3, pp. 364–367, Mar. 2023, https://doi.org/10.1177/21501351231156109
-
A. S. K. Wong and S. W. C. Sin, “Short-term mechanical circulatory support (intra-aortic balloon pump, impella, extracorporeal membrane oxygenation, tandemheart): a review,” Annals of Translational Medicine, Vol. 8, No. 13, pp. 829–829, Jul. 2020, https://doi.org/10.21037/atm-20-2171
-
T. M. M. H. de By et al., “The European registry for patients with mechanical circulatory support (EUROMACS): first annual report,” European Journal of Cardio-Thoracic Surgery, Vol. 47, No. 5, pp. 770–777, Mar. 2015, https://doi.org/10.1093/ejcts/ezv096
-
L. W. Miller et al., “Use of a continuous-flow device in patients awaiting heart transplantation,” New England Journal of Medicine, Vol. 357, No. 9, pp. 885–896, Aug. 2007, https://doi.org/10.1056/nejmoa067758
-
A. P. Levin et al., “Outcomes of contemporary mechanical circulatory support device configurations in patients with severe biventricular failure,” The Journal of Thoracic and Cardiovascular Surgery, Vol. 151, No. 2, pp. 530–535.e2, Feb. 2016, https://doi.org/10.1016/j.jtcvs.2015.10.019
-
M. B. Basir et al., “Mechanical circulatory support in acute myocardial infarction and cardiogenic shock: challenges and importance of randomized control trials,” Catheterization and Cardiovascular Interventions, Vol. 98, No. 7, pp. 1264–1274, Mar. 2021, https://doi.org/10.1002/ccd.29593
-
J. Soltes, D. Rob, P. Kavalkova, J. Bruthans, and J. Belohlavek, “Growing evidence for LV unloading in VA ECMO,” Journal of Clinical Medicine, Vol. 12, No. 18, p. 6069, Sep. 2023, https://doi.org/10.3390/jcm12186069
-
A. Sen et al., “Mechanical circulatory assist devices: A primer for critical care and emergency physicians,” Critical Care, Vol. 20, No. 1, Jun. 2016, https://doi.org/10.1186/s13054-016-1328-z
-
S. M. Ayub-Ferreira, “Executive summary – guidelines for mechanical circulatory support of the Brazilian society of cardiology,” Arquivos Brasileiros de Cardiologia, Jan. 2018, https://doi.org/10.5935/abc.20180126
-
J. J. Teuteberg et al., “The society of thoracic surgeons intermacs 2019 annual report: the changing landscape of devices and indications,” The Annals of Thoracic Surgery, Vol. 109, No. 3, pp. 649–660, Mar. 2020, https://doi.org/10.1016/j.athoracsur.2019.12.005
-
“Biventricular assist device (BiVAD).” BioRender Science Templates, https://www.biorender.com/template/biventricular-assist-device-bivad.
-
A. Schlagenhauf, J. Kalbhenn, U. Geisen, F. Beyersdorf, and B. Zieger, “Acquired von willebrand syndrome and platelet function defects during extracorporeal life support (mechanical circulatory support),” Hämostaseologie, Vol. 40, No. 2, pp. 221–225, May 2020, https://doi.org/10.1055/a-1150-2016
-
J. Han and D. Trumble, “Cardiac assist devices: early concepts, current technologies, and future innovations,” Bioengineering, Vol. 6, No. 1, p. 18, Feb. 2019, https://doi.org/10.3390/bioengineering6010018
-
J. Yaung, F. A. Arabia, and M. Nurok, “Perioperative care of the patient with the total artificial heart,” Anesthesia and Analgesia, Vol. 124, No. 5, pp. 1412–1422, May 2017, https://doi.org/10.1213/ane.0000000000001851
-
F. Nestler, A. P. Bradley, S. J. Wilson, D. L. Timms, O. H. Frazier, and W. E. Cohn, “A hybrid mock circulation loop for a total artificial heart,” Artificial Organs, Vol. 38, No. 9, pp. 775–782, Sep. 2014, https://doi.org/10.1111/aor.12380
-
M. K. Brockhaus et al., “Downsizing of a pulsatile total artificial heart-the effect on hemolysis,” ASAIO Journal, Vol. 68, No. 1, pp. 34–40, Mar. 2021, https://doi.org/10.1097/mat.0000000000001415
-
J. G. Copeland et al., “Experience with more than 100 total artificial heart implants,” The Journal of Thoracic and Cardiovascular Surgery, Vol. 143, No. 3, pp. 727–734, Mar. 2012, https://doi.org/10.1016/j.jtcvs.2011.12.002
-
P.-E. Noly, W. Ben Ali, Y. Lamarche, and M. Carrier, “Status, indications, and use of cardiac replacement therapy in the era of multimodal mechanical approaches to circulatory support: a scoping review,” Canadian Journal of Cardiology, Vol. 36, No. 2, pp. 261–269, Feb. 2020, https://doi.org/10.1016/j.cjca.2019.11.027
-
S. F. Perrodin, O. Muller, F. Gronchi, L. Liaudet, R. Hullin, and M. Kirsch, “Extracorporeal total artificial heart as bailout surgery,” Journal of Cardiac Surgery, Vol. 32, No. 3, pp. 222–228, Feb. 2017, https://doi.org/10.1111/jocs.13110
-
U. Richez et al., “Hemocompatibility and safety of the carmat total artifical heart hybrid membrane,” Heliyon, Vol. 5, No. 12, p. e02914, Dec. 2019, https://doi.org/10.1016/j.heliyon.2019.e02914
-
J. Bornoff et al., “Fluid-structure interaction modelling of a positive-displacement total artificial heart,” Scientific Reports, Vol. 13, No. 1, Apr. 2023, https://doi.org/10.1038/s41598-023-32141-2
-
G. Luraghi et al., “A numerical investigation to evaluate the washout of blood compartments in a total artificial heart,” Artificial Organs, Vol. 44, No. 9, pp. 976–986, Jun. 2020, https://doi.org/10.1111/aor.13717
-
D. Timms, J. Fraser, M. Hayne, J. Dunning, K. Mcneil, and M. Pearcy, “The bivacor rotary biventricular assist device: concept and in vitro investigation,” Artificial Organs, Vol. 32, No. 10, pp. 816–819, Oct. 2008, https://doi.org/10.1111/j.1525-1594.2008.00633.x
-
S. Emmanuel et al., “Anatomical human fitting of the bivacor total artificial heart,” Artificial Organs, Vol. 46, No. 1, pp. 50–56, Oct. 2021, https://doi.org/10.1111/aor.14077
-
E. Acome et al., “Hydraulically amplified self-healing electrostatic actuators with muscle-like performance,” Science, Vol. 359, No. 6371, pp. 61–65, Jan. 2018, https://doi.org/10.1126/science.aao6139
-
H. Yang, C. Zhang, B. Chen, Z. Wang, Y. Xu, and R. Xiao, “Bioinspired design of stimuli-responsive artificial muscles with multiple actuation modes,” Smart Materials and Structures, Vol. 32, No. 8, p. 085023, Aug. 2023, https://doi.org/10.1088/1361-665x/ace4a9
-
A. Weymann et al., “Artificial muscles and soft robotic devices for treatment of end‐stage heart failure,” Advanced Materials, Vol. 35, No. 19, Mar. 2023, https://doi.org/10.1002/adma.202207390
-
E. T. Roche et al., “Soft robotic sleeve supports heart function,” Science Translational Medicine, Vol. 9, No. 373, Jan. 2017, https://doi.org/10.1126/scitranslmed.aaf3925
-
J. F. Gummert, A. Haverich, J. D. Schmitto, E. Potapov, R. Schramm, and V. Falk, “Permanent implantable cardiac support systems,” Deutsches Ärzteblatt International, Vol. 116, pp. 843–848, Dec. 2019, https://doi.org/10.3238/arztebl.2019.0843
-
S. E. A. Felix et al., “The role of long-term mechanical circulatory support in patients with advanced heart failure,” Netherlands Heart Journal, Vol. 28, No. S1, pp. 115–121, Aug. 2020, https://doi.org/10.1007/s12471-020-01449-3
-
C. S. Rihal et al., “2015 SCAI/ACC/HFSA/STS clinical expert consensus statement on the use of percutaneous mechanical circulatory support devices in cardiovascular care (endorsed by the American Heart Association, the Cardiological Society of India, and Sociedad Latino Americana de Cardiologia Intervencion; affirmation of value by the Canadian Association of Interventional Cardiology-Association Canadienne de Cardiologie d’intervention),” Journal of Cardiac Failure, Vol. 21, No. 6, pp. 499–518, Jun. 2015, https://doi.org/10.1016/j.cardfail.2015.03.002
-
N. Aissaoui et al., “Understanding left ventricular assist devices,” Blood Purification, Vol. 46, No. 4, pp. 292–300, Oct. 2018, https://doi.org/10.1159/000491872
-
M. G. Crespo‐Leiro et al., “Advanced heart failure: a position statement of the heart failure association of the european society of cardiology,” European Journal of Heart Failure, Vol. 20, No. 11, pp. 1505–1535, Jul. 2018, https://doi.org/10.1002/ejhf.1236
-
A. S. Varshney et al., “Use of temporary mechanical circulatory support for management of cardiogenic shock before and after the united network for organ sharing donor heart allocation system changes,” JAMA Cardiology, Vol. 5, No. 6, p. 703, Jun. 2020, https://doi.org/10.1001/jamacardio.2020.0692
-
M. Abiragi et al., “Temporary mechanical circulatory support in patients with cardiogenic shock: clinical characteristics and outcomes,” Journal of Clinical Medicine, Vol. 12, No. 4, p. 1622, Feb. 2023, https://doi.org/10.3390/jcm12041622
-
A. Kilic et al., “Evolving trends in adult heart transplant with the 2018 heart allocation policy change,” JAMA Cardiology, Vol. 6, No. 2, p. 159, Feb. 2021, https://doi.org/10.1001/jamacardio.2020.4909
-
Y. Xia et al., “Outcomes of heart transplant recipients bridged with percutaneous versus durable left ventricular assist devices,” Clinical Transplantation, Vol. 37, No. 4, Feb. 2023, https://doi.org/10.1111/ctr.14904
-
C. Grzyb, D. Du, and N. Nair, “Artificial intelligence approaches for predicting the risks of durable mechanical circulatory support therapy and cardiac transplantation,” Journal of Clinical Medicine, Vol. 13, No. 7, p. 2076, Apr. 2024, https://doi.org/10.3390/jcm13072076
-
J. Libiseller-Egger et al., “Deep learning-derived cardiovascular age shares a genetic basis with other cardiac phenotypes,” Scientific Reports, Vol. 12, No. 1, Dec. 2022, https://doi.org/10.1038/s41598-022-27254-z
-
C.-M. Liu et al., “Artificial intelligence-enabled model for early detection of left ventricular hypertrophy and mortality prediction in young to middle-aged adults,” Circulation: Cardiovascular Quality and Outcomes, Vol. 15, No. 8, Aug. 2022, https://doi.org/10.1161/circoutcomes.121.008360
-
K. Shameer, K. W. Johnson, B. S. Glicksberg, J. T. Dudley, and P. P. Sengupta, “Machine learning in cardiovascular medicine: are we there yet?,” Heart, Vol. 104, No. 14, pp. 1156–1164, Jan. 2018, https://doi.org/10.1136/heartjnl-2017-311198
-
S. Shu, J. Ren, and J. Song, “Clinical application of machine learning-based artificial intelligence in the diagnosis, prediction, and classification of cardiovascular diseases,” Circulation Journal, Vol. 85, No. 9, pp. 1416–1425, Aug. 2021, https://doi.org/10.1253/circj.cj-20-1121
-
M. van Smeden et al., “Critical appraisal of artificial intelligence-based prediction models for cardiovascular disease,” European Heart Journal, Vol. 43, No. 31, pp. 2921–2930, Aug. 2022, https://doi.org/10.1093/eurheartj/ehac238
-
M. Chiarito, L. Luceri, A. Oliva, G. Stefanini, and G. Condorelli, “Artificial intelligence and cardiovascular risk prediction: all that glitters is not gold,” European Cardiology Review, Vol. 17, Dec. 2022, https://doi.org/10.15420/ecr.2022.11
-
M. Abdekhoda and F. Ranjbaran, “Artificial intelligence applications in decision making for disease management,” Research Square, Jul. 2023, https://doi.org/10.21203/rs.3.rs-3110088/v1
-
S. M. Lauritsen et al., “Explainable artificial intelligence model to predict acute critical illness from electronic health records,” arXiv:1912.01266, Jan. 2019, https://doi.org/10.48550/arxiv.1912.01266
-
R. A. Beaupré, O. H. Frazier, and J. A. Morgan, “Total artificial heart implantation as a bridge to transplantation: a viable model for the future?,” Expert Review of Medical Devices, Vol. 15, No. 10, pp. 701–706, Oct. 2018, https://doi.org/10.1080/17434440.2018.1524294
-
C. Iadecola and J. Anrather, “The immunology of stroke: from mechanisms to translation,” Nature Medicine, Vol. 17, No. 7, pp. 796–808, Jul. 2011, https://doi.org/10.1038/nm.2399
-
A. A. Levesque et al., “Development of multidisciplinary anticoagulation management guidelines for patients receiving durable mechanical circulatory support,” Clinical and Applied Thrombosis/Hemostasis, Vol. 25, p. 107602961983736, Jan. 2019, https://doi.org/10.1177/1076029619837362
-
R. Kanji, C. Vandenbriele, D. R. J. Arachchillage, S. Price, and D. A. Gorog, “Optimal tests to minimise bleeding and ischaemic complications in patients on extracorporeal membrane oxygenation,” Thrombosis and Haemostasis, Vol. 122, No. 4, pp. 480–491, Jun. 2021, https://doi.org/10.1055/a-1508-8230
-
K. Fukamachi et al., “An innovative, sensorless, pulsatile, continuous-flow total artificial heart: device design and initial in vitro study,” The Journal of Heart and Lung Transplantation, Vol. 29, No. 1, pp. 13–20, Jan. 2010, https://doi.org/10.1016/j.healun.2009.05.034
-
C. Fox et al., “Hybrid continuous‐flow total artificial heart,” Artificial Organs, Vol. 42, No. 5, pp. 500–509, Jan. 2018, https://doi.org/10.1111/aor.13080
-
S. G. Chopski, W. B. Moskowitz, R. M. Stevens, and A. L. Throckmorton, “Mechanical circulatory support devices for pediatric patients with congenital heart disease,” Artificial Organs, Vol. 41, No. 1, pp. E1–E14, Nov. 2016, https://doi.org/10.1111/aor.12760
About this article
The authors have not disclosed any funding.
Author would like to express sincere gratitude to Prof. Ismail Lazoğlu, (Division of Mechanical Engineering, Faculty of Engineering at Koç University, Istanbul, Turkey) for his invaluable mentorship during the Koç University Highschool Research Program on “The Development of an Artificial Heart Pump and Left Ventricular Assist Device” affiliated with Koç University Manufacturing and Automation Research Center that I attended in 2023 Summer and to, Prof. Selim İsbir, MD, (Department of Cardiovascular Surgery, Yeditepe University Hospital, Istanbul, Turkey) for his teaching about the current management of CHF and the contemporary utilization of MCS devices and his guidance throughout the writing process.
The datasets generated during and/or analyzed during the current study are available from the corresponding author on reasonable request.
The authors declare that they have no conflict of interest.