Abstract
In order to reveal the mechanism of the chain disaster caused by the collapse of high-altitude dangerous rock bodies in high-altitude open-pit mines and the formation of debris flows, field survey method was used to clarify the morphology, range, and scale of the high-altitude dangerous rock bodies and debris flows. Combined with geological data of open-pit mines and mining technical conditions, the formation mechanism of high-altitude dangerous rock bodies and the causes of debris flow formation were studied. The laws of chain disaster evolution and disaster patterns were revealed. The research results showed that (1) the formation of the collapse-debris flow chain disaster is due to the coupling effect of internal poor engineering geological conditions and external factors such as rainfall, groundwater, freeze-thaw, and blasting vibration. The collapse mode of the dangerous rock body is tensile-crack and tipping, shear-sliding, and tensile-crack and seating. (2) The collapse disaster of high-altitude dangerous rock bodies in open-pit mines triggered the formation of debris flows by multi-level landslides in the open-pit mines. The overall evolution process of the chain disaster is “local landslide in the pit - collapse of high-altitude dangerous rock bodies - multi-level landslide debris flow in the pit - burying the pit”. (3) The two-dimensional finite element numerical simulation analysis shows that the safety factor of the slope of the open-pit mine is 1.06. The slope is in an unstable state, verifying the results of the collapse of high-altitude dangerous rock bodies on the slope and the formation of landslide debris flows. The research results can provide references for the measures to break the chain and control measures of the chain disaster caused by the collapse of mountain slopes - debris flows in high-altitude and high-altitude areas.
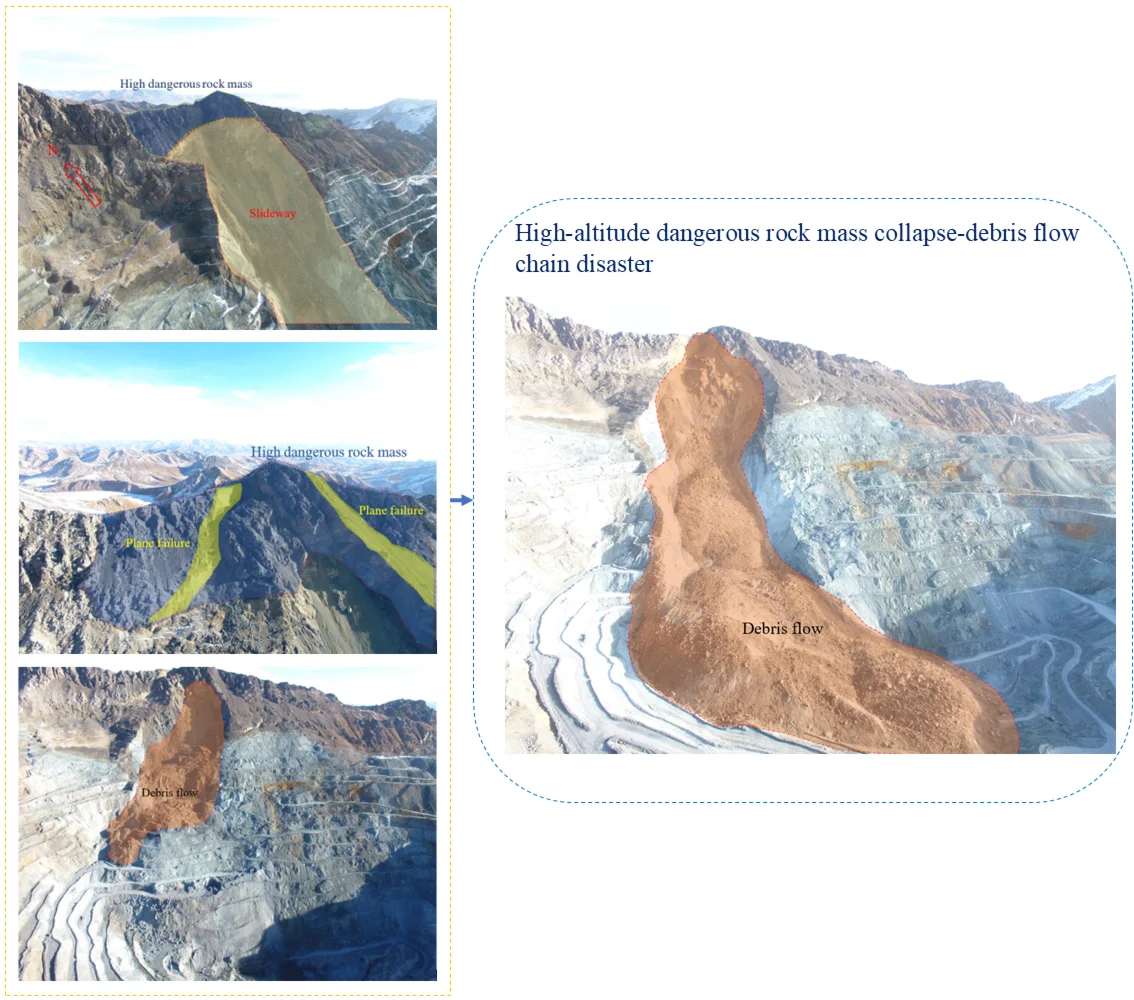
Highlights
- the formation of the collapse-debris flow chain disaster is due to the coupling effect of internal poor engineering geological conditions and external factors such as rainfall, groundwater, freeze-thaw, and blasting vibration.
- The collapse mode of the dangerous rock body is tensile-crack and tipping, shear-sliding, and tensile-crack and seating.
- The collapse disaster of high-altitude dangerous rock bodies in open-pit mines triggered the formation of debris flows by multi-level landslides in the open-pit mines.
- The overall evolution process of the chain disaster is "local landslide in the pit - collapse of high-altitude dangerous rock bodies - multi-level landslide debris flow in the pit - burying the pit".
1. Introduction
The collapse of high mountain rock bodies triggering debris flow chain disasters is characterized by strong destructive power, wide impact range, and difficulty in early prediction, and has always been one of the hot and difficult issues in the field of geological disasters [1-7].
Domestic and international cases of rock body collapse causing debris flow major disasters occur from time to time, bringing significant impact on social economy and people’s life safety [8-10]. At present, the study on the mechanism of high mountain rock body collapse in high-altitude open-pit mines triggering debris flow chain disasters is not in-depth, and it is urgently needed to fully understand the initiation mechanism, formation reasons, and scale of debris flow caused by the collapse of high mountain rock bodies in open-pit mines, which is of great significance for controlling the chain disasters of rock body collapse-debris flow, and achieving the prevention of geological disasters and the containment of major disaster accidents.
Chain disasters refer to a process where a natural disaster event occurs and subsequently triggers one or a series of secondary disasters, with the mechanisms of disaster formation involving both natural and anthropogenic factors [11-13]. The collapse-debris flow chain disaster is a chain disaster effect caused by the collapse disaster triggering a debris flow disaster. Currently, many scholars have conducted research on the causes and evolution mechanisms of collapse-debris flow chain disasters [14-17]. Gong et al. through field investigations, summarized the distribution patterns and developmental characteristics of collapses in the study area, and the collapse disaster has a typical chain evolution pattern of collapse-debris flow-river blockage, mainly controlled by factors such as freeze-thaw and topography. The giant collapse-debris flow disaster chain is mainly triggered by earthquakes, revealing the formation mechanism and evolution pattern of the collapse-debris flow disaster chain [18]. Shan et al. took the sudden collapse-debris flow geological disaster in the rear of the Wanguo Jizhen in Hanyuan County, Sichuan Province, as a case study, and through field geological investigations and theoretical calculations, concluded that the ancient accumulation body of Erman Mountain might become unstable and form a debris flow, and the loose material accumulated along the "7.27" in the Da Gou basin might trigger a large-scale debris flow again, posing a threat to Wanguo Jizhen [19]. Li et al. took the Tangjiashan landslide as a case study, and used numerical analysis and on-site monitoring research methods to carry out a stability study of the weathered loose accumulation above the Tangjiashan barrier body, revealing the “domino” chain disaster mechanism of earthquake-induced landslide → barrier lake → breach → flood [20]. Wan et al. based on the high-position chain geological disasters along the Jiali fault zone in Tibet, such as the Yigong Zangbu, Parlung Zangbu, and the downstream of the Yarlung Zangbo River causing regional disaster chains, used field research methods to summarize and analyze the disaster formation pattern of high-position chain geological disasters along the Jiali fault zone, revealing the disaster-gestation mechanism of high-position chain geological disasters involving multiple aspects such as geological structure and earthquakes, topography and water systems, glaciers, and meteorology [21]. Gao et al. through field site investigations, combined with multi-temporal satellite images and UAV data, took the Ze Long Nong Gully as an example, and clarified that the high-position ice-rock collapse disaster chain goes through four stages of ice-rock collapse, debris flow movement, accumulation blockage, and flood breach, forming a high-position long-distance geological disaster chain, and showing complex dynamic effects during the movement process, including impact disintegration effect, loose body arching effect, dynamic erosion effect, and debris accumulation effect [22]. Liu et al. took the high-position long-distance collapse-debris flow-mudslide disaster chain in Tiejiang Bay, Hongya County, Sichuan Province, on April 5, 2021, as an example, analyzed the evolution process of the large amount of rock blocks falling and impacting and loading the lower slope rock-soil body to form a landslide disaster chain, and used the RAMMS software to numerically simulate and analyze the debris flow movement process, and delineated its influence range under different rainfall frequencies [23].
Relying on specific engineering disaster cases as the research background, field investigation methods (such as manual exploration, drone scanning, and 3D laser scanning) were used to investigate and analyze the morphology, range, and scale of high-altitude and high-cold open-pit mines' high-risk rock bodies and debris flows, to clarify the characteristics of the high-risk rock bodies. Combining geological data of open-pit mines and mining technical conditions, the formation mechanism of high-risk rock bodies and the causes of debris flow formation were studied, revealing the chain disaster mechanism of collapse-debris flow in high-altitude and high-cold open-pit mines. The research results can provide references for the measures to break the chain and control the chain disasters of mountain slope collapse-debris flow in high-altitude and high-cold regions.
2. Geological conditions of the mining area
2.1. Terrain and landforms in the mining area
The mining area is located on the southwest slope of the high mountain area in the Snow Mountain range. The mountain range runs from northwest to southeast, with the terrain being higher in the northeast and lower in the southwest, with a slope gradient of 21-25°, and an elevation ranging from 4784-3980 m, with a relative height of more than 800 m.
At present, the mining area is in the mid-low mountain landscape unit at high altitude. Due to open-pit mining, the original terrain has been completely altered, forming mining pits. The bottom elevation of the mining pit is 4220 m. The ore body is distributed in a northwest direction. The lower part of the mining pit is 170-240 m above the valley, and the upper boundary of the mining pit is 300-320 m above the watershed. The topography and geomorphology of the mining area are shown in Fig. 1.
Fig. 1Landform and topography of the mining area [24]
![Landform and topography of the mining area [24]](https://static-01.extrica.com/articles/24594/24594-img1.jpg)
2.2. Engineering geological conditions
The research background case of the open-pit mine divides the slope rock mass into five engineering geological rock groups [25] based on the characteristics of the rock lithology, structural type, rock mass strength, hardness of the rock blocks, and weathering, and alteration.
Massive to fractured hard rock group: Mainly composed of hard rocks with massive to mosaic structures, representative lithologies include ores and peridotite, carbonated serpentinites, moderately weathered to slightly weathered, with high rock mass strength and good stability.
Massive to fractured semi-hard rock group: Mainly composed of semi-hard rocks with mosaic to fractured structures, representative lithologies include serpentinite, carbonated brecciated serpentinite, and serpentinite intercalated with peridotite, strongly weathered to moderately weathered, with rock mass strength controlled by the degree of weathering and schistosity.
Fractured to disintegrated loose rock group: Mainly composed of fractured to disintegrated serpentinite, representative lithologies include schistose serpentinite, gray-green in color, with a mineral composition dominated by fibrous and leafy serpentine. The slope rock mass is moderately weathered to strongly weathered, with highly developed schistosity, loose and fragmented, very low strength, and the lithology is soft with poor stability.
Massive to bedded sandstone group: The interbedded sandstone group with alternating hard and soft layers is mainly composed of massive to bedded sandstones, representative lithologies include metamorphic sandstone, sandstone. The slope rock mass is in a state of hardness to semi-hardness, with high strength, brittleness, and well-developed fractures, susceptible to blasting vibrations, with a single bench prone to the formation of dangerous rock bodies. Distributed in the northern part of the mining field and around the ore body.
Soft disintegrated residual slope deposit rock group: Mainly composed of residual slope debris soil with a disintegrated structure, widely distributed around the current mining field, on the top of the slope, in the mountain gullies, and at the foot of the slope, see Fig. 2 for the geological profile of the open-pit mine collapse-debris flow disaster.
The engineering geological rock groups that make up the slope body are diverse, ranging from the massive to fractured hard rock group to the soft disintegrated residual slope deposit rock group, etc., coupled with the destruction and disturbance of the rock mass caused by open-pit excavation and the induced re-distribution of rock mass stress, leading to varying degrees of engineering geological problems on the open-pit mine slope, such as: collapse of slope body (bench) steps, landslides of the slope, and potential collapse of dangerous rock bodies, etc., the complexity of the engineering geology of the mining area is of a medium type.
Fig. 2Geological profile of open-pit mining collapse [24]
![Geological profile of open-pit mining collapse [24]](https://static-01.extrica.com/articles/24594/24594-img2.jpg)
2.3. Hydrogeologic condition
The atmospheric precipitation in the mining area is relatively abundant. The atmospheric precipitation that falls on the surface forms surface runoff, which is divided by the surface watershed and flows southwestward along the slope, eventually draining to the surface water in the mountainous concave area at the foot of the southwest slope, forming a river that flows from west to east. The lowest erosional base level of the mining area is at an elevation of 3951 m, which serves as the water collection center for both surface water and groundwater in the mining area.
Based on the conditions of groundwater burial, the nature of rock layer porosity, the lithological unit, rock lithological combination, and the water-rich nature of the rock layers, the groundwater in the mining area is divided into six types: the Quaternary aquifer, the carbonaceous and iron-bearing siliceous slate aquifer, the gossan aquifer, the marble aquifer, the amphibolite aquifer, the ultrabasic rock aquifer, and the frozen layer aquifer. According to the requirements of relevant specifications, the mining area is classified as a deposit with simple hydrogeological conditions.
2.4. Rock strength parameters
Through conducting indoor rock mechanics freeze-thaw experiments, the strength parameters of slope rocks under freeze-thaw conditions were obtained. Since there is a size difference between the indoor rock mechanics test samples and the rock mass in engineering, it is necessary to transform the rock mechanics parameters into engineering-scale rock mass mechanics parameters. Common empirical methods include the RQD classification method, the CSIR classification method for jointed rock masses, the Q system classification method, the GSI classification method, and the geotechnical specification method for processing the physical and mechanical test parameters of mine rocks [26]. On the basis of the above methods, statistical knowledge is adopted. Based on the results of multiple methods, select the results with a 95 % confidence interval as the rock mechanics parameters. The comprehensively obtained strength of the slope rock mass after the weakening effect of freeze-thaw is shown in Table 1.
Table 1Rock mass strength by freeze-thaw action
Rock | Unit weight (kN/m3) | Naturalness | Freeze thaw weakening | ||
CmCm (MPa) | φm (°) | Ci (MPa) | φi(°) | ||
Slate | 27.3 | 0.33 | 34 | 0.26 | 28.35 |
Serpentinite | 25.4 | 0.18 | 30 | 0.07 | 11.42 |
Foliated serpentinite | 25.0 | 0.03 | 29 | 0.01 | 10.98 |
Carbonated serpentinite | 26.2 | 0.28 | 31 | 0.10 | 11.88 |
Peridotite | 26.7 | 0.55 | 32 | 0.44 | 26.58 |
Ore body | 46.7 | 0.659 | 43 | 0.53 | 36.72 |
3. Characteristics of landslide debris flow disasters
3.1. High level dangerous rock mass collapse disaster
The high-risk rock mass is located on the slope of the mining area from an elevation of 4476 m to the mountain top at 4734.88 m, with a slope height of 258 m. There is a large crack at the top of the slope, which is about 38 m long, 1.4-6.7 m wide, and 3-4.3 m deep.
The structural surfaces of the dangerous rock mass include: (1) The east side slope sinking surface of the landslide body (below 4520 m): 308 m length, with a sinking of about 10 m, the sinking surface strikes at 236° with a dip of 61°. The northern part of the sinking surface is slate, and the southern part is serpentinite. There are multiple crack phenomena on the southern platform of the sinking surface. (2) The mountain top crack: 75 m length, about 3 m wide, and about 1-3 m depth, the sinking surface strikes at 259° with a dip of 86°. The rock type of the crack is slate, with some sandstone intercalations, and there are 4 small cracks on the east side of the crack, 6-11 m length and about 0.1-0.2 m width. (3) The mountain top landslide body sinking surface: 129 m length (with the western landslide surface being 82 m long and the eastern sinking surface being 47 m length), the western landslide surface is about 20 m wide, the landslide surface strikes at 181° with a dip of 70°; the eastern sinking surface sinks about 1-2.5 m, the sinking surface strikes at 190° with a dip of 58°. (4) The west side sinking surface of the landslide body: 167 m length, connected to the mountain top landslide surface, with a sinking of about 10 m, the sinking surface strikes at 195° with a dip of 60°, the northern part of the sinking surface is marble, and the southern part is slate, with multiple crack phenomena on the southern platform of the sinking surface.
Multiple structural surfaces form a combined damage to the top of the dangerous rock mass, severely reducing the stability of the entire rock mass. There are two main collapse directions: (1) Along the vertical slope line direction of the mining area is 218°. (2) Along the direction perpendicular to the crack (in this direction, it is affected by the water pressure and frost heave force inside the crack), it is 259°.
The main rock types that make up this dangerous rock mass include: granite, siliceous slate, marble, and amphibolite, etc. The rock exposed in the crack area is amphibolite, which is hard and brittle, belongs to the hard rock mass, plus the frequent blasting engineering activities in the mining area, the development of cracks, and the appearance of loose dangerous rock masses. The underlying rock layers are mainly schistose serpentinite, serpentinite, peridotite, etc., and their strength is generally lower than the strength of the overlying rock mass. The main types of rocks in the landslide area include sandy slate, serpentinite, schistose serpentinite, etc.
Affected by more than three sets of rock mass structural surfaces, the controlling rock mass structural surfaces include: the smooth surface strike of 181°∠70° that constitutes the upper west side of the current landslide body, the rock layer strike of 26°∠34°~15°∠60 ° controlled by the back wing of the regional anticline, and other structural surfaces such as joints and cracks within the region.
In the early stage of the mining area, a landslide occurred, forming a landslide trough (Fig. 3), the stability of the top of the dangerous rock mass is very important, and the possible collapse forms mainly include: the tilting type driven by water pressure and frost heave force along the direction perpendicular to the crack; the sliding type affected by the cutting of the structural surface along the slope direction; and the seat collapse type controlled by the strength of the underlying low-strength schistose serpentinite.
Fig. 3Sliding groove formed after the landslide
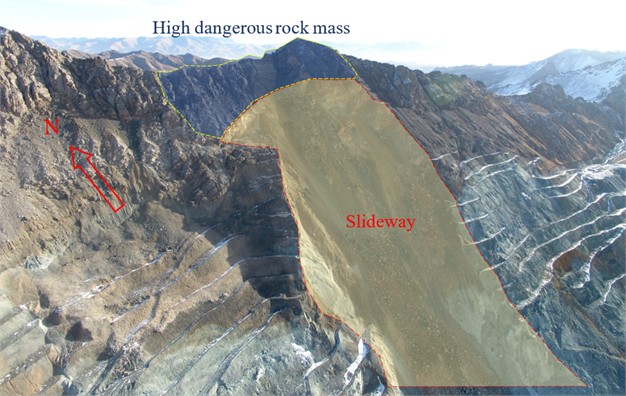
The high-altitude rock mass collapse disaster in the open-pit mining area is primarily located in the rock mass ranging from 4476 to 4734 m in elevation, which exhibits the following characteristics:
The rock mass is severely weathered, with its structural surfaces progressively deteriorating due to factors such as rainfall, snowmelt, and freeze-thaw cycles, leading to severe weathering of the rock mass.
The rock mass has well-developed structural surfaces, which experience a reduction in strength due to weathering, rainfall, snowmelt, freeze-thaw cycles, and other factors.
The dangerous rock mass is frequently disturbed, as the mining area uses blasting methods to excavate the ore body, causing disturbances to the dangerous rock mass.
The base of the dangerous rock mass has lost its support; the dangerous rock mass forms after a landslide in the mining area, leaving it suspended on the mountain top, devoid of the supporting function of the underlying rock mass.
The potential modes of failure for the dangerous rock mass primarily consist of: (1) The sliding type, controlled by the structural plane (195°∠51°) and the smooth surface after sliding (Plane failure as depicted in Fig. 4 and Fig. 5), which is also the direction of slide for the collapsed sliding body that has already occurred, representing the main direction for the future sliding of the dangerous rock mass. (2) The tilting type along the vertical fissure direction, mainly driven by the hydrostatic pressure and frost heave force within the fissure. (3) The seat collapse type, controlled by the tensile and compressive strengths of the lower phyllosilicate serpentinite, indicating the presence of a physically and mechanically weak layer at the base. Under the influence of gravity and other forces, the rock mass squeezes the weak layer, causing the rear part of the rock mass to tear and separate from the parent rock, resulting in the formation of a dangerous rock mass. The strength of the phyllosilicate serpentinite at the base of the dangerous rock mass in the open-pit mining area is insufficient to bear the weight of the overlying 258 m high dangerous rock mass, which includes granite, siliceous slate, marble, and amphibolite.
Fig. 4Before local dangerous rock collapse
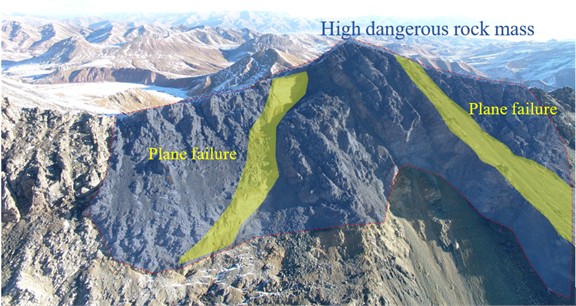
Fig. 5After local dangerous rock collapse
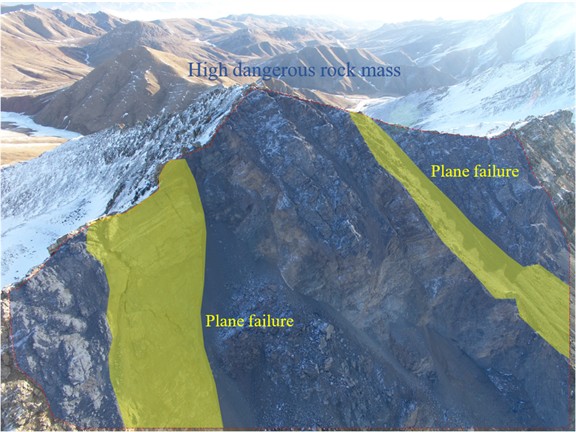
3.2. Open-pit step debris flow disaster
The open-pit mining bench collapse disaster has formed a landslide area between 4230 m and 4650 m, which can be divided into two stages of debris flow disasters in terms of time and space.
The first stage of debris flow disaster the first stage of the debris flow disaster is the formation of a local landslide on the northern slope of the open-pit mining area. The main rock type at the top of the northern slope is serpentinite, which is highly susceptible to softening upon contact with water, is prone to weathering, and has poor rock quality. Under the influence of external factors such as rainfall, freeze-thaw cycles, and blasting vibrations, the serpentinite on the northern slope deteriorates and crumbles, forming a local landslide. Due to the high degree of fragmentation of the serpentinite, the rock mass disintegrates during the sliding process, forming smaller rock fragments, thus creating a debris flow disaster. The debris flow formed by the local landslide, under the blocking effect of the open-pit bench, has not yet impacted the bottom of the open-pit mining area (Fig. 6).
The debris flow formed by the local landslide has had a certain impact on the stability of the bottom steps of the open-pit mining area. The debris is concentrated and piled up on the steps, imposing a load on them, and there is a potential trend of landslide on the platform of the lower open-pit mining area slope. Similarly, the local landslide has left the rock mass at the top of the slope in a suspended state, with the rock mass losing its lower support, forming a dangerous rock mass at the top. Under the action of gravity, it is prone to collapse disasters. The first stage of the debris flow disaster triggered the second stage of the debris flow disaster and formed a dangerous rock mass at the top of the slope.
Fig. 6First stage of debris flow disaster in the mining area
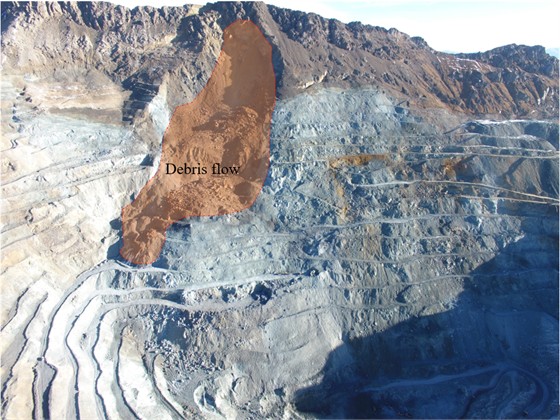
The second phase of the debris flow disaster the second phase of the debris flow disaster is the formation of a through-sliding landslide on the northern slope of the open-pit mining area. The main rock type at the top of the northern slope is serpentinite, which is highly susceptible to softening upon contact with water, is prone to weathering, and has poor rock quality. Field investigations and historical data analysis indicate that after the first phase of the debris flow disaster, the high-altitude dangerous rock mass at the top of the slope, having lost its lower support, experienced a collapse disaster. The high-altitude rock mass impacted the lower slope platform and the debris on it, causing the lower bench rock mass to gradually lose stability. At the same time, under the influence of external factors such as rainfall, freeze-thaw cycles, and blasting vibrations, the serpentinite on the northern slope deteriorated and crumbled, forming a through-sliding landslide that slid from the top of the slope to the bottom. During the sliding process, the rock mass disintegrated, mainly consisting of fragmented rock, thus forming the debris flow disaster in the open-pit mining area (Fig. 7). Using 3D laser scanning combined with 3DMINE software, the volume of the debris flow was measured to be approximately 200×104 m3. The formation of the second phase of the debris flow disaster is the result of the coupling of the collapse of the high-altitude dangerous rock mass with the first phase of the debris flow disaster and the combined action of external factors.
Fig. 7Second stage of debris flow disaster in the mining area
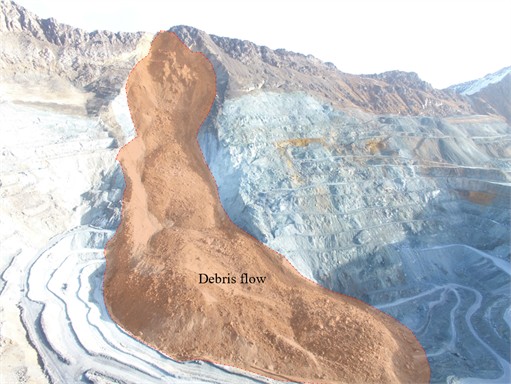
4. Collapse debris flow chain effect
4.1. Causes of landslide and collapse disasters
4.1.1. Poor engineering geological conditions
The schistose serpentinite, distributed in a northwest-trending zone on the west and north sides of the mining area, is locally banded around the ore body and within the serpentinite. Its rock nature is soft, and stability is poor. The slope rock mass is moderately to strongly weathered, with highly developed schistosity, which tends to be in the opposite direction of the mining slope, loose and fragmented, with very low strength, having a fractured to disintegrated structure. Some areas have schistosity that is consistent with the slope attitude, and the slope's shear resistance decreases year by year. Single benches are prone to instability and sliding along structural planes, while multiple benches are susceptible to arc-shaped failure due to water, vibration, freeze-thaw effects, and the influence of mining at the foot of the slope. Serpentinite intercalated with peridotite, distributed outside the mining boundary line on the northeast side of the mining area, is banded in a northwest direction, dipping to the northeast, and exhibits varying degrees of serpentinization with a blocky structure. The rock mass near the slope is mainly strongly weathered, with developed fractures and a fractured structure. The rock is semi-hard, has low strength, poor stability, and is prone to instability [27].
For the slate, marble, and granite that make up the upper part of the landslide body slope, fractures cut to form a fractured to mosaic structural rock mass. This part of the rock mass is strong and brittle, belonging to hard rock mass. In addition, the mining area is frequently subjected to blasting engineering activities, with developed fractures. The deformation and compression of the slope, as well as the degradation of rock mass strength due to freeze-thaw, result in loose high-altitude dangerous rock masses.
4.1.2. The role of groundwater in slopes
Water is one of the most important external factors affecting the stability of slopes. Groundwater in slopes can reduce the mechanical strength of the rock mass, softening the argillaceous rocks formed by weathering and alteration. Moreover, the seepage pressure of groundwater can increase the sliding force and decrease the resistance to sliding. Atmospheric precipitation not only forms surface flow on the slope, eroding and soaking the slope rock mass, reducing the strength of structural planes, but also infiltrates the slope to replenish groundwater. The mining area is located on the northern edge of the Qinghai-Tibet Plateau, with a cold and variable climate, and rainfall is relatively concentrated in the season, mainly from May to September.
The groundwater in the mountain body of the mining area is divided into two types: frozen layer water and bedrock fracture water. The frozen layer water is distributed in the mid-high mountain areas above 4200m in the mining area. The groundwater is mainly stored in the rock mass structural fractures. Serpentinite is prone to softening when in contact with water. Due to the scouring, softening, and freeze-thaw ice splitting effects of atmospheric rainfall (snow), bedrock fracture water, and upper underground seepage water, the original geological structure is prone to damage, leading to a reduction in the slope's anti-sliding (shear) capacity. In summary, the schistose serpentinite, serpentinite, and other rock masses that make up the mining slope are prone to softening when in contact with water. The action of groundwater has an important impact on the stability of the slope.
4.1.3. Freeze-thaw action
Due to the mining area being located in a high-cold, high-altitude area with permafrost, the freeze-thaw action here is very intense. Water in the pores or fractures of the rock mass expands in volume when it freezes into ice, deepening and widening the rock mass fractures. When the ice melts, water seeps further into the interior of the rock mass along the enlarged fractures, and the amount of water may also increase, freezing into ice again. This frequent process of freezing and melting continuously expands the fractures, leading to the disintegration of the rock mass [28].
The mining area is also a seasonal cold region. Under the freeze-thaw environmental conditions of the cold region, the change in temperature has an impact on the stability of the slope that cannot be ignored. Since rocks are naturally damaged materials, freezing causes water to move towards the frozen zone, forming a multi-phase damaged medium of water, ice, and rock inside it. The formation and development of ice bodies in rocks generate huge freezing expansion forces. This uneven freezing expansion force and deformation have an important impact on the stability of the slope. The temperature difference between morning and evening and the four seasons in the cold region causes freeze-thaw cycles, and the freezing expansion deformation affects the rock mass structure. During the melting process, this deformation cannot be completely recovered, further intensifying the shrinkage, damage, and cracking of the rock mass, as well as a series of physical and mechanical alternating changes, which are very unfavorable to the stability of the slope engineering.
4.1.4. Influence of blasting vibration
Blasting is frequent during the mining and stripping process of the mine. On the one hand, blasting vibration directly damages the rock mass in the blasting area. On the other hand, it causes post-blast tensile fractures in the surrounding rock mass of the blasting area, increasing the degree of fragmentation of the rock mass and the degree of opening of the fractures. Moreover, the dynamic load effect of blasting vibration can increase the shear stress and tensile stress of the rock mass, directly leading to a reduction in slope stability. This factor is an important human-induced factor affecting slope stability.
4.2. Formation mechanism of landslide debris flow chain disaster
Relying on a specific case of high-altitude and cold open-pit mine high-risk rock mass collapse-debris flow disaster as the background, a study on the chain disaster effect of collapse-debris flow was carried out. The slope rock mass of high-altitude and cold open-pit mines, compared to rock masses in ordinary areas, is long-term subjected to freeze-thaw and phase change cycles, making it highly susceptible to accelerated weathering and fragmentation under the action of external forces such as water erosion and ice cracking, directly leading to a reduction in rock mass strength and ultimately forming unfavorable engineering geological conditions. The blasting vibration of open-pit mining and the erosive action of groundwater affect the already weakened slope rock mass (such as serpentinite), leading to easy instability of the slope and forming the initial local landslide disaster on the northern slope of the open-pit mine. The main reason for the formation of the initial landslide is the unfavorable engineering geological conditions as the internal cause, while rainfall, groundwater, freeze-thaw, and blasting vibration as external causes have accelerated the formation of the landslide.
After the initial landslide disaster on the northern slope of the open-pit mine was formed, the rock mass at the top of the slope did not collapse and slide with the landslide body, forming a high-altitude dangerous rock mass. Similar to the formation mechanism of the initial landslide disaster, under the combined action of internal causes such as unfavorable engineering geological conditions, and external causes such as rainfall, groundwater, freeze-thaw, and blasting vibration, the high-altitude dangerous rock mass gradually collapsed. The collapsed body impacted the lower slope platform at high speed, which on the one hand, exacerbated the formation of slope platform instability, and on the other hand, imposed a load on the slope platform, ultimately leading to the instability and landslide of the lower slope, with the rock mass breaking and loosening, forming a debris flow disaster that buried the open-pit mine. The overall evolution process of the chain disaster can be described as “local landslide in the mining area – high-altitude dangerous rock mass collapse - multi-bench landslide debris flow in the mining area – burial of the mining area”.
5. Numerical simulation analysis of chain disasters
5.1. Finite element analysis model
Using the Canadian geotechnical engineering analysis software Phase2 8.0, an analysis of the stability of the high-altitude dangerous rock mass on the slope of the open-pit mining area was conducted. Based on the engineering geological characteristics of the open-pit mining slope, a geological profile that can represent the typical geological and topographical conditions of the high-altitude collapse body was selected as the finite element analysis model. The model includes slate, schistose serpentinite, serpentinite, and peridotite, as shown in Fig. 8. A plane strain analysis was performed on the slope model to obtain results such as the overall displacement of the mining slope, plastic zone, and safety factor, and to study the sliding mode of the dangerous rock mass [29].
Fig. 8Finite element analysis model of dangerous rock mass
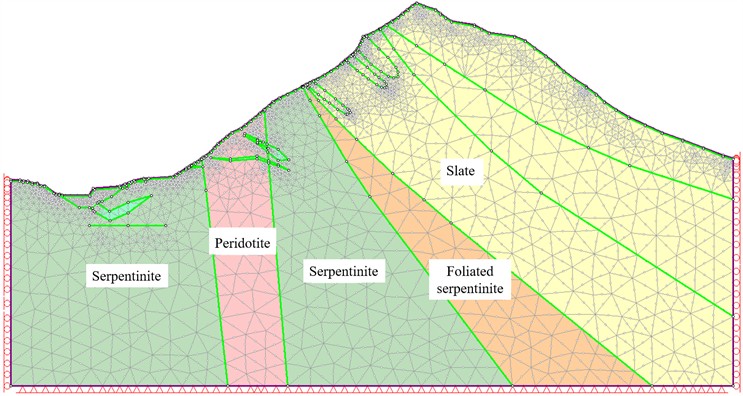
When judging the stability of dangerous rocks based on the stability coefficient of dangerous rocks, according to the requirements of the specification. The engineering safety level of the open-pit mining slope is between level two and level three, and considering the actual situation such as the mining time of the open pit, the stability evaluation indicators for the dangerous rock mass were determined to be: Safety factor < 1.00 unstable; 1.00 ≤ Safety factor < 1.15 marginally stable; 1.15 ≤ Safety factor < 1.30 basically stable; Safety factor ≥ 1.30 stable
5.2. Results analysis of numerical simulation
A finite element stability analysis was conducted on the slope of the open-pit mine containing high-altitude dangerous rock masses, and results such as the safety factor, plastic zone, and displacement of the slope were obtained, as shown in Fig. 9, Fig. 10 and Fig. 11.
Fig. 9Slope safety factor and potential sliding surface
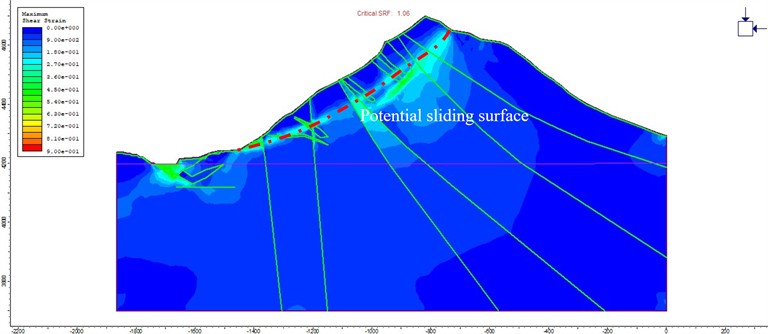
Fig. 10Distribution of plastic zone on slope
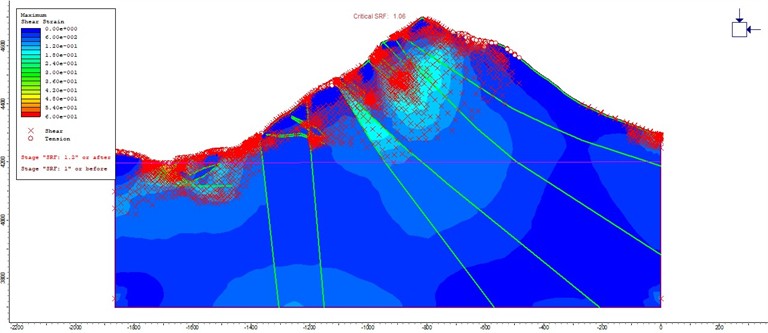
The overall safety factor of the slope is 1.06, indicating a marginally stable state, which is prone to dangerous rock mass collapse disasters under the influence of external factors (heavy rainfall, earthquakes, blasting disturbances, etc.). The areas where plastic deformation occurs on the slope are mainly at the top of the slope where the dangerous rock mass is located, the slope face, and potential sliding surfaces, indicating that the slope has the potential for collapse-debris flow chain disasters. The finite element analysis shows that the maximum deformation displacement of the slope reaches 30 meters, indicating that the slope has already experienced a collapse and landslide disaster. Multiple numerical simulation results confirm that there is a risk of collapse of the high-altitude dangerous rock mass in the open-pit mine, and that the collapse can trigger a landslide in the open-pit mine, leading to a debris flow disaster. The overall evolution process of the chain disaster can be described as “local landslide in the mining area – collapse of high-altitude dangerous rock mass - multi-bench landslide debris flow in the mining area – burial of the mining area”.
Fig. 11Slope displacement cloud map
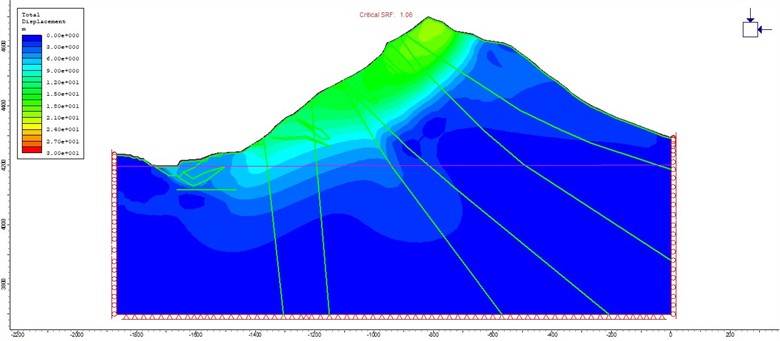
6. Conclusions
Based on field investigation methods, the morphology, range, and scale of high-altitude dangerous rock masses and debris flows in a high-altitude and cold Open-pit Mine were investigated and analyzed to clarify the characteristics of the high-altitude dangerous rock masses. Combined with geological data of open-pit mines and mining technical conditions, the formation mechanism of high-altitude dangerous rock masses and the causes of debris flow formation were studied, and the following conclusions were drawn:
1) The rock properties and the cutting of structural surfaces lead to poor engineering geological conditions. The reasons for the formation of landslide-collapse-debris flow chain disasters are the internal causes of poor engineering geological conditions, coupled with external factors such as rainfall, groundwater, freeze-thaw, and blasting vibrations, which gradually led to the occurrence of landslide-collapse-debris flow chain disasters. There are several collapse modes of dangerous rock masses, including tensile cracking and tilting, shear and sliding, and tensile cracking and seating.
2) High-altitude dangerous rock masses in open-pit mines undergo collapse disasters, and under the impact of collapse, multi-bench landslides in the open-pit mine form debris flow disasters. The overall evolution process of the chain disaster can be described as “local landslide in the mining area – collapse of high-altitude dangerous rock mass - multi-bench landslide debris flow in the mining area – burial of the mining area”.
3) Two-dimensional finite element numerical simulation analysis shows that the safety factor of the northern slope of the open-pit mine is 1.06. Combined with the results of the plastic zone and displacement, it can be determined that the slope is in a state of insufficient stability, which verifies the results of the collapse of the high-altitude dangerous rock mass on the slope and the formation of landslide debris flow.
References
-
A. Brinton, D. C. Diehl, T. Townsend, K. Y. Deliz Quiñones, and M. M. Lichtenstein, “Trees, trash, and hurricanes: The case study of Puerto Rico and vegetative disaster debris management after Hurricanes Irma and Maria,” SSRN Electronic Journal, Vol. 82, Jan. 2022, https://doi.org/10.2139/ssrn.4127853
-
X. Fan et al., “Earthquake‐induced chains of geologic hazards: patterns, mechanisms, and impacts,” Reviews of Geophysics, Vol. 57, No. 2, pp. 421–503, Jun. 2019, https://doi.org/10.1029/2018rg000626
-
Y. Tie et al., “Research on the pattern of typical geohazard chains in the southwest mountainous region, China,” Journal of Geomechanics, Vol. 28, No. 6, pp. 1071–1080, 2022.
-
F. Gao and X. Gao, “On geological hazard chain research in China in recent 20 years,” (in Chinese), Journal of Geological Hazards and Environment, Vol. 35, No. 1, pp. 55–62, 2024.
-
W. Zhou et al., “Combining rainfall-induced shallow landslides and subsequent debris flows for hazard chain prediction,” CATENA, Vol. 213, p. 106199, Jun. 2022, https://doi.org/10.1016/j.catena.2022.106199
-
T. Zhang et al., “Characteristics and dynamic analysis of the February 2021 long-runout disaster chain triggered by massive rock and ice avalanche at Chamoli, Indian Himalaya,” Journal of Rock Mechanics and Geotechnical Engineering, Vol. 15, No. 2, pp. 296–308, Feb. 2023, https://doi.org/10.1016/j.jrmge.2022.04.003
-
L. Zhu et al., “Analyzing the multi-hazard chain induced by a debris flow in Xiaojinchuan River, Sichuan, China,” Engineering Geology, Vol. 293, p. 106280, Nov. 2021, https://doi.org/10.1016/j.enggeo.2021.106280
-
X. Wang et al., “Formation mechanism of a disaster chain in Loess Plateau: a case study of the Pucheng County disaster chain on August 10, 2023, in Shaanxi Province, China,” Engineering Geology, Vol. 331, p. 107463, Mar. 2024, https://doi.org/10.1016/j.enggeo.2024.107463
-
B. Ding, Y. Meng, and X. Pei, “Engineering geological investigation and assessment on rockfall hazard of one project in Nepal,” Journal of Engineering Geology, Vol. 29, No. 2, pp. 554–563, 2021, https://doi.org/10.13544/j.cnki.jeg.2020-046
-
F. Wang, Q. Ren, X. Jiang, A. Jiang, C. Zhao, and W. Liu, “Engineering geology and subsidence mechanism of a mountain surface in the Daliang lead-zinc ore mine in China,” Bulletin of Engineering Geology and the Environment, Vol. 81, No. 11, p. 488, Oct. 2022, https://doi.org/10.1007/s10064-022-02983-7
-
Z. Xue, C. Xu, H. Gao, and Y. Huang, “Disaster chain thinking improves the capabilities of disaster prevention, mitigation, and relief in China,” Natural Hazards Research, Vol. 4, No. 2, pp. 320–323, Jun. 2024, https://doi.org/10.1016/j.nhres.2023.11.013
-
W. Liu et al., “Analysis of natural disaster chain and chain-cutting disaster mitigation mode,” (in Chinese), Chinese Journal of Rock Mechanics and Engineering, Vol. 25, pp. 2675–2681, 2006.
-
S. Xiao et al., “Shape characteristics of evolvement in chain-styled phases of disasters,” (in Chinese), Chinese Journal of Rock Mechanics and Engineering, Vol. 25, pp. 2629–2633, 2006.
-
Y. Liu, “The mechanism of disasters induced by coupling of endogenic and exogenic geological processes in the Yarlung Zangbo river from Gongga county to Jiacha county,” (in Chinese), Chengdu University of Technology, 2022.
-
S. Xu, Y. Yin, and A. Xing, “Characteristic analysis of the Nayong rock avalanche’s kinematics based on seismic signals,” (in Chinese), The Chinese Journal of Geological Hazard and Control, Vol. 31, No. 2, pp. 1–8, 2020.
-
Z. Wei, “Study on the initiation mechanism and kinematic mechanism of collapsed clastic grain flow,” (in Chinese), Chongqing Jiao Tong University, Chongqing Jiao Tong University, 2020.
-
G. Wang et al., “Simulation of the process of the Jiguanling rock avalanche in Wulong of Chongqing,” (in Chinese), Hydrogeology and Engineering Geology, Vol. 41, No. 5, pp. 101–106, 2014.
-
C. Gong et al., “Characteristics of collapse development and geohazard chain model in the Dongcuoqu Basin, eastern Tibet,” (in Chinese), Drilling Engineering, Vol. 50, No. 5, 2023.
-
S. Shan, X. Wang, and T. Li, “Debris flow prediction and comprehensive treatment of "7.27" post-disaster of Wangong town, Hanyuan county, Sichuan province,” (in Chinese), The Chinese Journal of Geological Hazard and Control, Vol. 23, No. 4, pp. 6–10, 2012.
-
S. Li et al., “Study of geological origin mechanism of Tangjiashan landslide and entire stability of landslide dam,” (in Chinese), Chinese Journal of Rock Mechanics and Engineering, Vol. 29, pp. 2908–2915, 2010.
-
J. Wan, H. Chu, and B. Li, “Characteristics, types, main causes and development of high-position geohazard chains along the Jiali fault zone, Tibet, China,” (in Chinese), The Chinese Journal of Geological Hazard and Control, Vol. 32, No. 3, pp. 51–60, 2021.
-
S. Y. Y. Gao et al., “Dynamic characteristics of the rock-ice avalanche disaster chain in the Zelongnong basin, Yarlung Zangbo river canyon region,” Journal of Engineering Geology, Vol. 32, No. 3, pp. 996–1009, 2024, https://doi.org/10.13544/j.cnki.jeg.2023-0163
-
Liu Xin et al., “Research on evolution process and impact range prediction of high level remote collapse and landslide-debris flow disaster chain-taking the “4·5” Tiejiangwan geological disaster chain in Hongya county, Sichuan province as an example,” (in Chinese), Journal of Jilin University (Earth Science Edition), Vol. 53, No. 6, pp. 1799–1811, 2023.
-
A. Huang, “Slope stability analysis for an open-pit mine,” (in Chinese), Dalian University of Technology, 2016.
-
F. Wang, Q. Ren, X. Jiang, J. Niu, and B. Chen, “Engineering geology and mechanism of multiple landslides in a large open-pit mine: the case of the copper mine in Qinghai Province, China,” Bulletin of Engineering Geology and the Environment, Vol. 82, No. 4, Mar. 2023, https://doi.org/10.1007/s10064-023-03186-4
-
E. Hoek and E. T. Brown, “The Hoek-Brown failure criterion and GSI – 2018 edition,” Journal of Rock Mechanics and Geotechnical Engineering, Vol. 11, No. 3, pp. 445–463, Jun. 2019, https://doi.org/10.1016/j.jrmge.2018.08.001
-
F. Wang et al., “Strata movement law based on progressive caving of the hanging wall: a case of study in Chaganaobao Iron-Zinc Mine,” Arabian Journal of Geosciences, Vol. 13, No. 21, pp. 1155–1170, Oct. 2020, https://doi.org/10.1007/s12517-020-06112-0
-
D. Zhao, “Study on slope landslide evolution mechanisms of the derni copper mine under the freeze-thaw action,” (in Chinese), Metal Mine, Vol. 7, pp. 165–170, 2016.
-
Z. Ma et al., “Research on formation conditions and numerical simulation of multiple landslides in Derni Copper Mine,” (in Chinese), Journal of Disaster Prevention and Reduction, Vol. 39, No. 3, pp. 1–6, 2023.
About this article
This work was supported by the Natural Sciences Funding Project of Hunan Province (2024JJ6110, 2024JJ8049), Application Basic Research and Soft Science Research Plan of Yiyang City (2024YR02), and aid program for Science and Technology Innovative Research Team in Higher Educational Institutions of Hunan Province.
The datasets generated during and/or analyzed during the current study are available from the corresponding author on reasonable request.
Wang Feifei wrote the initial draft (including substantive translation). Jiang Anmin carried out the site investigation.
The authors declare that they have no conflict of interest.